Abstract
This pilot study investigated the biomechanical properties of prefabricated, vascularized bioartificial bone grafts, which may provide an alternative bone source for the restoration of segmental osseous defects. Vascularized bioartificial bone grafts comprise an artificial customized scaffold made of beta-tricalcium phosphate. Bone formation along the prefabricated scaffold is induced by autogenous cancellous bone. Vascularization of the bone graft is provided by the host’s vascular system. Within 6 months, a mammalian bioreactor (sheep were used in the present study) creates heterotopic vascularized bioartificial bone grafts of a predetermined anatomical shape, which can be harvested for reconstructing osseous defects. The bioartificial bone grafts in this study contained up to 25% bone tissue, as shown by histomorphometric analysis and computed tomography. Moreover, unconfined compression tests revealed that the constructs had mechanical characteristics similar to those of ovine cancellous bone. Therefore, this method could be applied to generate vascularized prefabricated bone substitutes for critical-size defects.
The restoration of critical-size bone defects that are not compensated by endogenous bone regeneration remains a challenge in regenerative medicine, particularly in the craniomaxillofacial region. A critical-size defect is classified as the smallest size of defect that will not heal completely during the natural lifetime of an animal.
To date, autologous bone grafts, which are transferred as either vascularized or non-vascularized tissue, are the most effective method for reconstruction. In particular, when grafted as avascularized tissue or in combination with synthetic scaffolds, the incorporated autogenous osteogenic cells and growth factors provide the main source of ossification. Hence, regeneration occurs along the natural or synthetic scaffold with osteoinductive and osteoconductive properties. Although this approach yields high rates of incorporation and initial revascularization, the disadvantages include donor site morbidity, limited availability, and plasticity.
In contrast to orthopaedic medicine, reconstruction for facial skeleton defects poses two major challenges. First, because rehabilitation with dental implants is the principle purpose of osseous reconstruction of the maxilla and mandible, the grafted bone will inevitably be exposed to the paranasal sinus and the oral cavity and its microflora, albeit indirectly. Second, the complexity of the three-dimensional (3D) shape of the facial skeleton, maxillo-mandibular unit, and masticatory tasks require bone grafts with high plasticity and mechanical strength. In craniomaxillofacial reconstruction, these defects are primarily treated using either vascularized or non-vascularized flaps from the fibula, scapula, or iliac crest. However, the bone quality, structure, and 3D contour do not fully meet the requirements for a functional and aesthetic outcome true to the original. Thus, secondary augmentations and soft tissue corrections are often necessary, particularly in cases where dental implants are placed. Computer-assisted surgery has recently revolutionized the field of reconstructive craniomaxillofacial surgery, and allogeneic patient-specific implants have already emerged as a standard procedure in many institutions.
Novel synthetic materials and surface coatings have recently been developed for bony reconstruction. In particular, synthetic calcium phosphate bone substitutes such as hydroxyapatite (HA) and beta-tricalcium phosphate (β-TCP) have received increasing attention due to the similarity of their chemical composition to bone and their ability to become resorbed whilst regenerating new bone. To date, the production of scaffolds that fully comply with all requirements remains challenging, and most in vitro attempts using synthetic materials have failed to engineer large viable and vascularized bone grafts. In particular, the mechanical properties of novel bone substitutes provide challenges for clinical application. Thus, innovative approaches are essential to generate viable individualized alternatives that meet the requirements for functional and aesthetic reconstruction of the facial skeleton.
In contrast to the classical in vitro tissue engineering approach, this study employed in vivo tissue engineering. Briefly, a mammalian bioreactor generates bioartificial bone grafts for osseous reconstruction, providing oxygen and nutrition through vascularization. Combining the principles of autogenous bone graft reconstruction with in vitro tissue engineering techniques, this approach uses an osteogenic cocktail provided by bone marrow aspirates to vitalize artificial scaffolds. Customized, prefabricated bone grafts, first described in pigs and humans by Terheyden et al., have been modified and developed further and are now reproducibly available in complex shapes. However, their biomechanical properties require evaluation before they can be applied routinely in humans. This study aimed to investigate the biomechanical characteristics of prefabricated, axially vascularized bioartificial bone grafts generated in a large mammalian bioreactor system.
Materials and methods
Animal experiments
The study used six female black-headed German sheep with an average weight of 69.3 kg. All of the animal experiments were performed under general anaesthesia in accordance with the requirements of the institutional ethics committee and the federal animal protection law (reference number 33.9-42502-04-08/1621). Following induction with diazepam (0.2 mg/kg) and ketamine hydrochloride (3–4 mg/kg Ursotamin; Rebopharm GmbH, Bocholt, Germany), general anaesthesia was maintained using an isoflurane–oxygen mixture (2.5 vol% in oxygen mixture, Isoba; Essex Pharma GmbH, Munich, Germany). Intra- and postoperative analgesia was achieved via the administration of fentanyl (0.005 mg/kg) and carprofen (4 mg/kg) (Sigma-Aldrich Chemie GmbH, Steinheim, Germany) and buprenorphine (10 mg/kg Buprenovet; Bayer Healthcare, Leverkusen, Germany). All of the animals also received perioperative antibiotics (cefquinome (2 mg/kg Cobactan 4.5%); Intervet GmbH, Unterschleißheim, Germany). The antibiotic regimen was continued for 1 week post-operation.
Prefabrication, implantation, and tissue harvesting
An arrangement of angulated cylinders was chosen in order to simulate the complexity of defects in the craniofacial skeleton, e.g. the mandibular angle. Two β-TCP cylinders (porosity 60–80%, pore size 100–500 μm), 14 mm in diameter and 25 mm in length (chronOS; Synthes, West Chester, PA, USA) and with a central perforation (7 mm in diameter), were assembled at a 30° angle and wrapped in a customized titanium cage (20 mm in diameter and 60 mm in length) formed using an autoclavable plastic template ( Fig. 1 A, B ). The prefabricated angulated constructs were implanted into the latissimus dorsi region of the experimental animals, using axial perfusion provided by the thoraco-dorsal trunk, as described previously. The space between the β-TCP cylinders and the titanium cage, as well as the central perforation, was filled with autogenous cancellous bone from the iliac crest ( Fig. 1 C, D).

After 6 months, the animals were sedated with diazepam (0.2 mg/kg) and euthanized with intravenous sodium pentobarbital (4500–6000 mg Release(R), WDT eG, Garbsen, Germany). The constructs were harvested along with healthy cortical and cancellous reference bone samples from the metacarpal, radial, and iliac crest regions of the sheep for comparative biomechanical analysis. Long bone samples were chosen as controls because of their similar cross-sectional area, as required for sufficient comparative compression testing. Following explantation, the titanium cage was removed and the constructs were prepared for immediate micro-computed tomography (micro-CT) ( Fig. 1 E, F).
Micro-computed tomography
Following sample harvesting and removal of the titanium cage, constructs, reference bone samples, and a β-TCP cylinder were scanned with a high-resolution peripheral quantitative computed tomography scanner (XtremeCT; Scanco Medical, Brüttisellen, Switzerland; 60 kVp, 901 μA, voxel size 41 μm, integration time 300 ms) for precise characterization of the samples. Initially a visual two-dimensional (2D) analysis was done using one longitudinal (middle position) and three cross-sectional (25%, 50%, 75%) slices. After contouring and determining threshold values for obviously different shaded regions (gauss sigma 0.8, gauss support 1.0; threshold values: β-TCP cylinder preoperative −1000 to 135 Hounsfield units (HU) for pores filled with air, 136–1000 HU for scaffold; constructs and reference bone 136–1000 HU; soft tissue in constructs 41–135 HU), the analysis was performed using the true 3D evaluation software (μCT Evaluation Program v. 6.0; Scanco Medical) for 3D and local 3D calculations. Using the same thresholds for all samples, the volume, surface (inner and outer area including the pores), bone and scaffold microstructure (trabecular number, separation, and thickness), and density were calculated. As the peripheral quantitative computed tomography (pQCT) was calibrated against a phantom for bone density measurements, the density was recorded in milligrams hydroxyapatite per cubic centimetre (mgHA/cm 3 ). For an additional evaluation of β-TCP cylinder remnants inside the constructs, the cylinder was differentiated visually from the bone using the parameters structure, shape, and position and accordingly contoured accurately in each slice. Then a new calculation was performed using the previous threshold. Representative 3D reconstructions of a single β-TCP cylinder pre-operation and post-explantation are shown in Fig. 2 .

Sample preparation for mechanical testing
Immediately after micro-CT, constructs and reference bone samples were prepared for mechanical testing. The test samples were cut into cylinders (height 8 mm) for comparative biomechanical testing using an Exakt cutting system (Exakt Advanced Technologies GmbH, Norderstedt, Germany). For accurate and parallel slicing of cylinders of precisely 8 mm, samples were fixed on a cover slip using a cold-polymerizing, three-component resin (Technovit 4000; Heraeus-Kulzer GmbH, Wehrheim, Germany), according to the manufacturer’s instructions. The off-cuts were selected for histological and histomorphometric analysis. The prepared samples were stored at −20 °C until biomechanical testing was performed.
Histology
The off-cuts remaining from cutting the samples were immediately fixed in 3.5% neutral buffered formalin. Samples were embedded in methylmethacrylate (MMA) and sectioned perpendicular to the cylinder axis using a modified inner-hole diamond saw. Standard light microscopy and histomorphometric analysis were performed on undecalcified slices of 30 μm in thickness, following surface staining with Alizarin–methylene blue. Digital images of each slide were obtained using a Zeiss AxioImager MI microscope fitted with an AxioCam MRc digital camera and AxioVision 4.5 software (Carl Zeiss, Oberkochen, Germany). The AxioVision module MosaiX was used to scan the whole specimen. Electronically created images of the entire cross-section of the constructs provided the basis for further analysis. The total bone area, residual scaffold area, and connective tissue area were quantified using Image Analysis Software 3.01 (Olympus Soft Imaging Solutions, Münster, Germany).
Compressive mechanical testing
A total of 23 unconfined compression tests were performed on six prefabricated, vascularized bioartificial bone grafts, and on eight cancellous and 13 cortical bone samples. These samples were chosen as controls because of their similar cross-sectional area, as required for sufficient comparative compression testing, and the fact that both bone qualities (cortical and cancellous bone) are used for craniofacial reconstruction. Prior to mechanical testing, the cross-sectional area of the test specimens was measured in square millimetres (mm 2 ) using a custom-built 2D laser scanner (MHH Research Workshop). Specimen height was measured at four arbitrary points around the cylinder, and a mean was calculated ( Fig. 3 ). The samples were tested under uniaxial compression using a Mini Bionix 858 material testing machine (MTS Systems, Minneapolis, MN, USA). This system allows for axial compression with a maximum load capacity of 15 kN and a displacement range of ±50 mm. The test rig consisted of a pivoting plate (upper grip) and a cylindrical stainless steel platform (lower grip), between which the specimens were compressed to failure. The ceramic crosshead of the actuator was fitted into the central socket of the pivoting plate to avoid misalignment that could place shear loads on the specimen. The test samples were centred on the lower grip on the load cell to avoid contact between the upper grip and the load cell. Each compression test was performed at a constant displacement rate of 10 μm/s without preconditioning. The crosshead was lowered to meet the socket of the upper grip and contact was established at a reading of 10–20 N. The test was stopped when the specimen was squeezed to failure or the load capacity exceeded 1 kN. A linear regression of the stress–strain curve, up to 30% of the strain range, was used to evaluate the linear uniaxial compressive modulus. Ultimate stress values were extracted from the curves.

Statistical analysis
Statistical analyses were performed using Sigma Plot 10 (Systat Software Inc., San Jose, CA, USA) and Microsoft Office Excel, Version 2010 (Microsoft Corporation, Redmond, WA, USA). The data were first tested for normal distribution using the Kolmogorov–Smirnov procedure. Normally distributed, independent values within the experimental groups were subjected to analysis of variance (ANOVA) followed by post hoc tests (Tukey). Comparisons between the experimental groups at particular points in time were performed using the Student t -test. Independent values that were not normally distributed were analyzed using Kruskal–Wallis one-way ANOVA followed by the Mann–Whitney U -test. For all analyses, P < 0.05 was considered significant.
Results
Solid bioartificial bone grafts
No implant infections or rejections were observed during the 6-month period, indicating high biocompatibility of the constructs. The constructs showed a solidity and stability comparable to that of bone. The outer contours of the constructs were preserved and wrapped in a vascularized soft tissue envelope. The original angulation of the constructs was conserved in most samples. Macroscopic examination following cutting showed that the originally implanted arteriovenous trunk could be identified in the cross-sectional views. The conjunctional area, where the two angulated cylinders of a single construct met, appeared to be interstratified with osseous tissue.
Outer and internal structure as revealed by micro-CT
For visual analysis, 2D and 3D images of both longitudinal and cross-sectional slices of the constructs were evaluated. After 6 months, the preformed, angulated shape of the constructs was still visible, and the conjunction was highly ossified. Close bone-to-scaffold contact remained, despite high degradation of the β-TCP cylinders. Bone-like structures were observed to have invaded and replaced the scaffold. The arteriovenous trunk could be identified in the central perforation of the cylinder. Representative 2D and 3D construct scans are shown in Fig. 4 .

A comparison of the basic pre-operation and post-explantation properties of a single β-TCP cylinder revealed decreased scaffold volume, surface-to-volume ratio, and scaffold density. The mean thickness and separation of the scaffold material was increased, whereas the number of scaffold trabeculae was decreased. However, in agreement with the properties of the prefabricated constructs, density was increased slightly compared to the preoperative β-TCP cylinder. The surface-to-volume-ratio was also increased, but it did not exceed the basic value. A density comparison showed that healthy autogenous cortical bone was considerably denser than prefabricated constructs. Although the mean thickness and separation of bone and scaffold material in the constructs also differed from those of healthy bone, a similar number of trabeculae could be observed. Taken together, after 6 months, the density, mean material thickness, and material separation of prefabricated constructs were increased to the disadvantage of trabecular number and surface-to-volume ratio. Thus, the surface area of the prefabricated constructs decreased in relation to their volume. By subtracting the soft tissue volume (79.13%) from the total volume of the construct, the average percentage of bone and scaffold was determined to be 20.86%. The basic characteristics and trabecular properties of the constructs in comparison to an original β-TCP cylinder and reference bone samples are summarized in Fig. 5 .
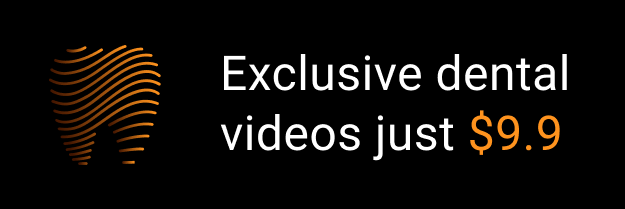