Introduction
An electromechanical device was used to experimentally characterize the movement of a single tooth within the periodontal ligament space. The force magnitude leading to the complete compression of the periodontal ligament is considered a critical force and is designated Fc. We investigated the effectiveness of the electromechanical device to repeatedly determine the critical force magnitude Fc.
Methods
The study comprised 12 tests conducted on 11 subjects. Alternating labial and lingual forces were applied to a maxillary incisor by the device. The resulting immediate intra-alveolar tooth displacement was recorded in real time. Data processing was used to determine the tooth mobility curve for 193 push-pull cycles. The critical force Fc was mathematically determined for both the labial and lingual displacements of the tooth.
Results
The tooth mobility curve could be characterized for all 12 tests. A total of 386 values of Fc were calculated for the 12 different teeth. Values of Fc for each test ranged from 10.47 to 20.18 g in the lingual direction, and from 12.56 to 21.72 g in the labial direction.
Conclusions
The electromechanical appliance was successful in repeatedly determining Fc in vivo. The ability to experimentally determine the extent of periodontal ligament compression at a given force magnitude could shed new light on the question of an optimal orthodontic force and open new avenues of orthodontic research and treatment.
Highlights
- •
An electromechanical device was used to characterize tooth movement in the PDL.
- •
Force magnitude to fully compress the PDL was designated a critical force (Fc).
- •
Data processing of in-vivo measurements allowed 386 values of Fc to be calculated.
- •
Mean magnitude of Fc to fully compress the PDL was consistently 10 to 22 g.
Tooth mobility refers to the temporary displacement of a tooth in its alveolar space when subjected to an externally applied force. Even though the term “tooth mobility” is more commonly used in the context of pathologic tooth mobility and the classification of periodontal disease, nonpathologic or physiologic tooth mobility was the focus of this study.
This intra-alveolar mobility of a tooth is largely facilitated by the viscoelastic properties of a healthy periodontal ligament (PDL), which is deformed under a combination of tensile, compressive, and shear forces when a force is applied to a tooth. The force-displacement curve depends on many factors: tooth and root sizes and geometry, PDL thickness, age and health of the patient, and direction and position of force application.
Previous studies have measured the physiologic tooth mobility of rats in vitro, have used numeric models to simulate the tooth mobility curve for human teeth, and have combined numeric and in-vitro methods to study the same in minipigs. Comparable tooth mobility curves have also been measured using a laser vibrometry approach in vitro and a magnetic sensor array in vivo. We used a specialized electromechanical device to measure the same tooth mobility curve for human teeth in vivo.
The tooth mobility curve is nonlinear and typically consists of the 2 clearly distinguishable phases of initial tooth mobility (ITM) and secondary tooth mobility (STM) as identified by Mühlemann. These 2 phases of tooth mobility have been confirmed by several further studies.
The force magnitude at which the immediate tooth mobility transitions from ITM to STM is designated Fc to indicate a critical force level. By fitting a straight line to each of the ITM and STM phases, the value of Fc can be mathematically determined. Fc could therefore provide an important reference point when discussing orthodontic forces.
Forces of magnitude exceeding Fc could be considered excessive, since they are more likely to fully compress the PDL. Knowing the value of Fc could prevent overloading the PDL and the related negative effects that will hinder tooth movement. Forces of magnitude below Fc would be less likely to cause disturbance of blood flow, discomfort, or focal hyalinization. As is the case for Fc, such focal hyalinizations are individually and locally determined because of irregularities in the PDL and bone morphology. Patient and case-specific local geometric irregularities will limit tooth mobility, thereby directly affecting the value of Fc.
The ability to measure which force magnitudes will cause partial compression of the PDL, and which will lead to a locally compressed PDL, could also shed new light on the concept of an optimal orthodontic force as first defined by Schwarz.
The tooth mobility curve is measured instantaneously and requires controlled forces to be applied for only short periods of time; hence, no significant tissue remodeling occurs. A clear differentiation should be made between tooth mobility and tooth movement; the latter is the result of the continued application of a force to a tooth and the resulting tissue remodeling.
The materials and methods described hereafter were used to determine the case and tooth specific values of Fc, and therefore the force magnitude at which the PDL is likely to be fully compressed, for 12 cases.
Material and methods
Institutional review board approval for this in-vivo study was obtained from the Pharma Ethics Committee of South Africa (reference, 160713422; protocol, OFT2016-01). Participants were invited to voluntarily take part in the study to determine the effectiveness of an electromechanical device in determining the critical force level F C . Each participant was briefed and the test procedure explained in detail, and written consent was obtained from each participant.
Participants enrolled in the study were between the ages of 12 and 30 years. They were not classified by age. Patient age is acknowledged to play a significant role in orthodontics; however, the objective of this study was to determine whether Fc could be determined in participants of different ages. No comparisons or conclusions were drawn with regard to participant age.
To ensure that the measured tooth mobility was nonpathologic, visual clinical inspections were done on each participant, confirming good oral hygiene, lack of gingivitis, no reddening or edema, and no observable loss of attachment.
Furthermore, subjects were considered only if they had at least 1 maxillary central or lateral incisor that did not make contact with any adjacent teeth to ensure that the force-displacement curve was measured for a single tooth only and was not affected by other teeth.
A total of 12 tests were conducted on 11 participants. The 2 tests conducted on the same participant were done on 2 different teeth (maxillary left central and lateral incisors), and the second test was done 3 days after the first. The first test was assumed to have no impact on the second.
See Video for the test procedure, available at: www.ajodo.org .
A dental impression of the maxilla was taken, and a plaster model was produced to replicate the patient’s dentition. One maxillary central or lateral incisor was identified as the target tooth. A suitable tooth needed to allow for free labiolingual mobility, without interfering or making contact with any adjacent teeth. A custom biteplate was produced that fitted tightly over the maxillary teeth, but left the target tooth exposed as shown in Figure 1 , A .

The electromechanical devices were constructed, calibrated, and supplied by Ortho Future Technologies, Windhoek, Namibia. Each device contained an actuator that can produce pushing and pulling forces, a force sensor, and a position sensor. The force sensor measures the force applied by, or applied to, the actuator tip. It is calibrated to an accuracy of 1 g or less at a minimum resolution of 0.5 g. The position sensor, which measures the displacement of the actuator tip, has a minimum accuracy of 11 μm over the entire operating range of 1.2 mm and a minimum resolution of 5 μm. The system is contained in a titanium housing that is 22 mm long and has a diameter of 6.3 mm.
Two such devices were arranged in parallel and fixed to the biteplate as shown in Figure 1 , allowing a combined force of ±70 g over a range of 1.2 mm. The actuator tips were positioned to engage with the target tooth and the axis of movement orientated labiolingually. The biteplate and attached devices were placed into the patient’s mouth and the biteplate fitted onto the dentition. The tip of the device was fixed to the target tooth with a small screw clamp that had been temporarily bonded to the tooth, to allow both pushing and pulling forces to be applied.
The 2 devices were connected to a microcontroller unit, which communicates wirelessly and in real time with user interface software (Ortho Future Technologies) on a computer. The user interface allowed the clinician to control either the position of the actuator tip or the force applied to a tooth by the actuator. The system allowed the clinician to observe both the displacement and the force in real time on the user interface as shown in Figure 1 , B .
The devices were programmed to apply a cyclic force to the target tooth. Alternating labial and lingual forces followed a triangular wave form with an amplitude of 60 g and a period of 110 seconds per cycle. The microcontroller controlled each device individually and ensured that the total force applied to the tooth followed the desired profile by measuring the applied force of each device and readjusting the actuator every 10 ms (100 times per second).
Each test consisted of 18 complete push-pull cycles. For each cycle, the force was increased from 0 to 60 g (labial direction), reduced and reversed to −60 g (lingual direction), and reduced back to 0 g. The total time for completing 18 such cycles was 33 minutes per test.
Data were sampled and recorded at a frequency of 20 Hz, and each data point included the force applied to the tooth and the relative displacement of the target tooth in the labiolingual direction. Each complete data set comprised 2200 data points per cycle and 39,600 data points per test.
After completion of the test protocol, the devices were detached from the target tooth and the biteplate removed from the patient’s mouth. Data were stored on the microcontroller unit during testing and upon completing the test were downloaded via the user interface and stored on a local computer hard drive for further processing.
As a control test, the biteplate with the devices was placed onto the plaster of paris model, and the same test conducted on the rigid model. This ensured that the majority of the displacement measured in vivo was the displacement relating to the compression of the PDL and not to the elasticity of the experimental setup. Displacements were small compared with the in-vivo test results and were typically found to account for 5% to 15% of the corresponding in-vivo displacement.
Statistical analysis
The data for each test were grouped into 18 smaller data sets of 2200 data points per cycle. Each cycle was classified according to the quality of the raw data and was given 1 of 4 ratings: A, raw data had no irregularities; B, raw data had 3 or fewer irregularities that did not significantly affect the shape of the measured curve; C, raw data had irregularities that did significantly affect the shape of the curve; and D, raw data were not suitable for analysis using the mathematical methods because of excessive irregularities.
Only cycles with a quality rating of A or B were selected for further data analysis, resulting in 193 cycles from the total of 216 that were recorded.
The average tooth displacement was calculated for each 1g interval over the range from −60 to +60 g. The resulting curve relates the tooth displacement (dependent variable) to the applied force (independent variable) and is called the tooth mobility curve. The result is shown by the black curve labeled “processed data” in Figure 2 .

The first derivative with respect to the applied force δx / δF was calculated, where x denotes the relative displacement of the tooth in micrometers, and F is the applied force in grams. The resulting curve is a measure of tooth mobility, expressed in units of micrometers per gram μ m / gm : in other words, the amount of displacement that is caused by every 1 g of change in the applied force.
To mathematically determine the point at which mobility of the target tooth changes from labial to lingual or vice versa, the second derivative with respect to F, δ 2 x / δF 2 , was calculated, and its intercept with the x-axis was determined to the nearest gram. The point on the tooth mobility curve that corresponds to the force value of the x-intercept is designated F C0 . The first and second derivative curves as well as F C0 are shown in Figure 2 .
Because it is the relative displacement vs applied force that is of interest, the data set of each cycle was then centered on F C0 . The results for all 18 cycles of a single test are presented in Figure 3 , A .

Thereafter, the tooth mobility curve was used to identify the ITM and STM phases for each cycle. This was achieved by fitting 2 straight-line segments to the data in each labial and lingual direction. Segmented linear regression and the method of least squares were used to fit the straight-line segments to the ITM and STM regions. The break point for the segmented regression was determined by maximizing the coefficient of determination R 2 for both line segments in the labial and lingual directions.
The break point for the segmented regression coincides with the intersection of the ITM and STM lines and is equal to Fc. Depending on the direction of displacement, the corresponding value of Fc is designated F C Lingual or F C Labial. The results of the data processing procedure are shown in Figure 4 . Here, Fc 0 is located at the origin and shows the transition between labial and lingual displacements. F C Lingual or F C Labial presents the transition from ITM to STM. This is the point at which localized compression of the PDL is expected to occur. The same results were obtained for 193 cycles using the described data-processing procedures.
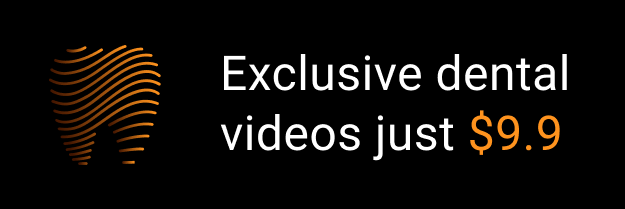