Abstract
Objectives
Collagen and elastin are two key structural proteins found in the extra-cellular matrices (ECMs) of most tissues, yet very little is known about the response of bone cells to elastin or its derivatives. Recently, we have designed and characterized a novel class of ECM-based composite scaffolds with collagen and a genetically engineered polymer, elastin-like polypeptide (ELP) and subsequently showed their superior mechanical properties and drug release characteristics compared to collagen scaffolds. The objective of this research was to evaluate osteoblast growth and expression on these composite scaffolds.
Methods
A thorough biochemical and morphological characterization was performed on MC3T3-E1 pre-osteoblast cells cultured on collagen and ELP–collagen scaffolds. Cell viability was assessed using a live/dead assay. Total DNA content of all cells present on various surfaces was quantified. Pre-osteoblast differentiation was assessed by measuring the alkaline phosphatase and osteocalcin production. Mineral deposition by the cultured cells was visualized using the Von Kossa stain.
Results
Our results showed that the ELP–collagen scaffolds were suitable substrates for cell culture that allowed MC3T3-E1 pre-osteoblast cell attachment, differentiation, and subsequent mineralization over a period of 3 weeks. The ELP–collagen scaffolds displayed equivalent biocompatibility and cell-interacting properties to those of the neat collagen scaffolds.
Significance
The novel ELP–collagen composite material may have future implications as a scaffold material for bone tissue engineering applications, for example, the treatment of alveolar bone loss.
1
Introduction
Over 1.5 million procedures were performed in the United States involving the treatment of fractures or joint replacements in 2007 . It is estimated that more than 3 million facial injuries occur each year in the United States . Loss of alveolar bone may occur due to trauma, tumor resection, periodontal infection, or tooth extraction and results in a bony defect that will not regenerate. Treatment options may include autografts, allografts, and xenografts . However, use of autografts may increase post-operative donor site morbidity . Allografts or xenografts may elicit immune response, implant rejection, or disease transmission . Thus, the need for bone tissue in numerous clinical situations and the limited availability of suitable bone grafts are the driving forces for the development of tissue engineering approaches to bone repair .
A scaffold used for bone tissue engineering should gel or set without being toxic to the cells and have appropriate chemical and physical properties that permit cell adhesion and differentiation. The suitable scaffolds should allow diffusion of nutrients and conform to the defect shape, while maintaining sufficient mechanical integrity . Bioceramics, polymers, and hydrogels have been used for such applications . Hydrogels are a class of highly hydrated three-dimensional, polymer materials (water content >30%, w/w). The retained high water content in hydrogels allows for efficient diffusion of nutrients contributing to their biocompatibility while maintaining a certain degree of structural integrity and elasticity . Hydrogels display mechanical and structural properties similar to the extra-cellular matrix (ECM) and may guide the cell migration and growth during organization processes . Hydrogels containing collagen, hyaluronic acid, fibrin, alginate, agarose, dextran, chitosan, and elastin-like polypeptides (ELPs) have been used as scaffolds for culturing cells due to their similarities with the ECM, self-assembling ability, long-term stability, and good biological performance .
Tissue engineering aims to restore both the structural integrity and the remodeling process of native ECM . Collagen and elastin are two key structural proteins found in the ECMs of most tissues and influence biological properties of engineered scaffolds . Collagen type I is predominantly found in skin, bone, tendons, and other connective tissues and has been used in a variety of physical forms (hydrogels, fibers, sheets, etc. ) for hemostasis, soft tissue augmentation, burn and wound dressing, and guided tissue regeneration for treatment of periodontal diseases . Collagen type I is known to cause differentiation of cultured bone progenitor cells into osteoblasts by specific cell membrane interactions and forms a major component of many FDA-approved synthetic grafts for maxillofacial use . However, collagen-based biomaterials suffer from poor mechanical properties for tissue engineering and are rapidly degraded by extra-cellular collagenases in vivo. Previous studies have shown that cross-linking of collagen or copolymerization with other polymers can have a significant influence in regulating the rate of degradation, enhancing the mechanical properties, and reducing antigenicity of collagen .
On the other hand, the extensive cross-linked structure of elastin makes it difficult to process it into biomaterials . Also, elastin from human or bovine sources is associated with the glycoprotein fibrillin that confers it a high propensity to calcify upon implantation . Hence, soluble and recombinant forms of elastin such as tropoelastin, alpha-elastin, and ELPs are frequently used to form cross-linked gels, fibers, or injectable scaffolds for tissue engineering and drug delivery . ELPs are biopolymers with the pentapeptide repeat Val–Pro–Gly–Xaa–Gly, where the Xaa, termed the guest residue, can be any of the natural amino acids except Pro . ELPs possess good biocompatibility with mechanical and viscoelastic properties similar to natural elastin. They are genetically engineered providing precise control on the structure and properties and exhibit an inverse phase transition behavior in response to changes in their solution conditions . We have demonstrated an effective release of model antibiotic doxycycline hyclate and model protein bovine serum albumin from novel ELP–collagen composite scaffolds for periodontal drug delivery applications (unpublished data). The objective of this research was to evaluate osteoblast attachment, growth, and subsequent differentiation on these ELP–collagen composite scaffolds.
2
Materials and methods
2.1
Expression and purification of ELP
The ELP had a primary sequence of H 2 N-MVSACRGPG-[VPGVG] 120 -WP-COOH, where A = alanine, C = cysteine, G = glycine, M = methionine, P = proline, R = arginine, S = serine, V = valine, and W = tryptophan and a molecular weight of 51,000 Da as measured by sodium dodecyl sulfate polyacrylamide gel electrophoresis (SDS-PAGE). Escherichia coli BLR(DE3) bacteria (Novagen, Madison, WI) having synthetic gene for this ELP were multiplied in nutrient broth at 37 °C for 24 h. The cells were then harvested and lysed by sonic disruption and cell debris were separated by centrifugation. The ELP was further purified by an inverse transition temperature ( T t ) method. This particular ELP had a T t of 32 °C. The purification method involved at least three repeated cycles of solubilization of ELP in phosphate buffered saline (PBS) at 4 °C, followed by precipitation and centrifugation at 40 °C. The ELP was then dissolved in deionized (DI) water and the solution was dialyzed against DI water using a regenerated cellulose membrane (Spectra/Por Dialysis Membrane, Spectrum Laboratories Inc., Rancho Dominguez, CA) to remove buffer salts. The ELP solution was analyzed by measuring the UV absorbance at 280 nm with a NDS 1000 spectrophotometer (Thermo Scientific, Wilmington, DE) and its concentration was determined by using a previously prepared calibration curve. The purified ELP was then lyophilized using a vacuum freeze dryer (Labconco Corporation, Kansas City, MO).
2.2
Preparation of collagen and ELP–collagen hydrogels
Collagen type I (Rat tail, 5 mg/mL) was purchased from GIBCO (Invitrogen, Carlsbad, CA). ELP was purified and freeze-dried using the purification process described earlier. The manufacturer’s protocol for collagen gel formation was followed to form collagen (8 mg) and ELP (25 mg)–collagen (8 mg) composite gels and the procedure was performed in a sterile hood to prevent contamination . In brief, sterile DI water (160 μL), 10× PBS (200 μL), and 1 N NaOH (40 μL) were mixed and ELP (25 mg) was dissolved in this solution. The collagen solution (1.6 ml; 5 mg/mL) was then mixed gently. The final solution was transferred to a 24-well tissue culture polystyrene (TCPS) plate (Costar, Corning Life Sciences, Lowell, MA) and incubated at 37 °C at 100% humidity for 24 h to form a gel. Neat collagen gels were fabricated using the same procedure for comparison.
2.3
Scanning electron microscopy (SEM)
The gels were frozen and then lyophilized for 12 h using the vacuum freeze dryer to obtain dry scaffolds for SEM imaging. Specimens were mounted on aluminum stubs and sputter-coated with gold for 2 min at 9 V. The samples were then scanned in the scanning electron microscope (SUPRA 40, Carl Zeiss, Thornwood, NY) at a voltage of 5 kV using both primary and secondary electron detectors. Images were recorded from at least 3 different areas on each of the gels at 200× and 1000× magnifications.
2.4
Mechanical testing
Detailed method is described elsewhere . In brief, 12 rectangular-strip shaped test specimens were pressure-cut using a tissue chopper (McIlwain Tissue Chopper, Mickle Laboratory Co. Ltd., Surrey, UK) and their dimensions were measured using Clemex image analysis microscope and software (Clemex Technologies, Quebec, Canada). The average thicknesses of the collagen and ELP–collagen specimens were 0.95 ± 0.08 mm and 1.73 ± 0.12 mm, respectively. The average widths of the collagen and ELP–collagen specimens were 2.33 ± 0.09 mm and 2.54 ± 0.21 mm, respectively. Uniaxial tensile testing was performed using ASTM D882 at an extension rate of 0.5 in/min (12.7 mm/min) on a Sintech 2/G Materials Testing System (MTS, Eden Prairie, MN) using a pre-load of 0.22 N . Values of tensile strength, Young’s modulus, and toughness were calculated using the MTS Testworks 4.0 software.
2.5
MC3T3-E1 cell culture
MC3T3-E1 mouse pre-osteoblastic cells (Subclone 4; American Type Culture Collection, Manassas, VA) were selected as they exhibit high levels of osteoblast differentiation and form a well mineralized ECM after 10 days. These cells are a good model for studying in vitro osteoblast differentiation and have been shown to behave similarly to the primary calvarial osteoblasts . MC3T3-E1 cells were maintained and expanded in alpha-modified Eagle’s minimum essential medium (α-MEM; Hyclone Laboratories, South Logan, UT), herein termed as “maintenance medium”, at 37 °C and 5% CO 2 . The medium was supplemented with 10% fetal bovine serum (FBS), 2 mM l -glutamine, 1% penicillin–streptomycin, and 2 mM sodium pyruvate (GIBCO, Invitrogen, Carlsbad, CA) with pH adjusted to 7.4. Cells were harvested using trypsin-EDTA at 70% confluence. The harvested cells were seeded onto collagen gels, ELP–collagen gels, and TCPS without the gels at a density of 50,000 cells/well in a 24-well TCPS plate. The TCPS surface was the control for all the subsequent assays and staining. After 24 h of culture, the cells were supplemented with 1 mL of differentiation medium containing 10 mM β-glycerophosphate and 50 μg/mL ascorbic acid.
2.6
Live/dead assay
Viability of the cells cultured on collagen and ELP–collagen hydrogels was assessed using a live/dead assay (Invitrogen, Carlsbad, CA) according to the manufacturer’s protocol. The assay was performed on day 14 for collagen gels, ELP–collagen gels, and TCPS surfaces cultured with MC3T3-E1 cells. In brief, the cells were washed with sterile PBS, incubated with live/dead reagents (4 μM EthD-1 and 2 μM calcein AM) for 25 min at 37 °C and then observed by epifluorescence microscopy using an Olympus IX 81 microscope equipped with a Slidebook image analysis software (Olympus America, Center Valley, PA).
2.7
Quantification of DNA
The MC3T3-E1 cells cultured on collagen and ELP–collagen gels were separated by incubating in 2 mL collagenase (2.68 mg/mL) mixed in a solution of 5 mM CaCl 2 in Krebs ringer buffer (KRB) for 20 min . The MC3T3-E1 cells cultured on the control TCPS surfaces were separated by trypsinization. The cells were then isolated from the culture media by micro-centrifugation at 2000 rpm, resuspended in 500 μL of PBS, and lysed on ice for 2 min of sonication at 10% amplitude using a Branson Sonifier. The cell lysates were analyzed using DNA assay (Sigma, St. Louis, MO). The assay utilizes a fluorescent dye that binds to DNA and increases the fluorescence significantly at 460 nm. DNA standards (0–35 μg/mL) and samples were incubated with the fluorescent dye in a 96-well TCPS plate (Costar, Corning Life Sciences, Lowell, MA) in the dark at room temperature for 30 min. The plate was read using an FLX-800 fluorescence plate reader (Biotek, Winooski, VT) using an excitation wavelength of 360 nm and emission wavelength of 460 nm. The DNA content for each of the wells was calculated using the equation of the standard curve adjusted with the values for the control standard (0 μg/mL DNA).
2.8
Alkaline phosphatase assay
A colorimetric kinetic determination of alkaline phosphatase (ALP) activity of the MC3T3-E1 cells was performed using QuantiChrom™ ALP assay Kit (DALP-250, BioAssay Systems, Hayward, CA) on day 14. In this method p-nitrophenyl phosphate (pNPP) is hydrolyzed by ALP into a yellow colored product, p-nitrophenolate (maximal absorbance at 405 nm). The rate of the reaction is directly proportional to the enzyme activity. The working solution for the assay was prepared by mixing 200 μL assay buffer, 5 μL Mg Acetate, and 2 μL pNPP liquid substrate. 25 μL of the cell lysate solution (as prepared earlier) was transferred into wells of a 96-well plate along with 150 μL of working solution. The plate was read at 405 nm ( t = 0) and again after 4 min ( t = 4 min) on an ELX-800 absorbance plate reader (Biotek, Winooski, VT) at an absorbance wavelength of 405 nm. The ALP activity was then calculated using the formula specified by the manufacturer as shown below:
ALP activity of sample ( μ mol / L min ) = ( O.D. SAMPLE t = 4 min − O.D. SAMPLE t = 0 min ) × 1000 × Reaction volume ( O.D. CALIBRATOR − O.D.H 2 O ) × Sample volume × time
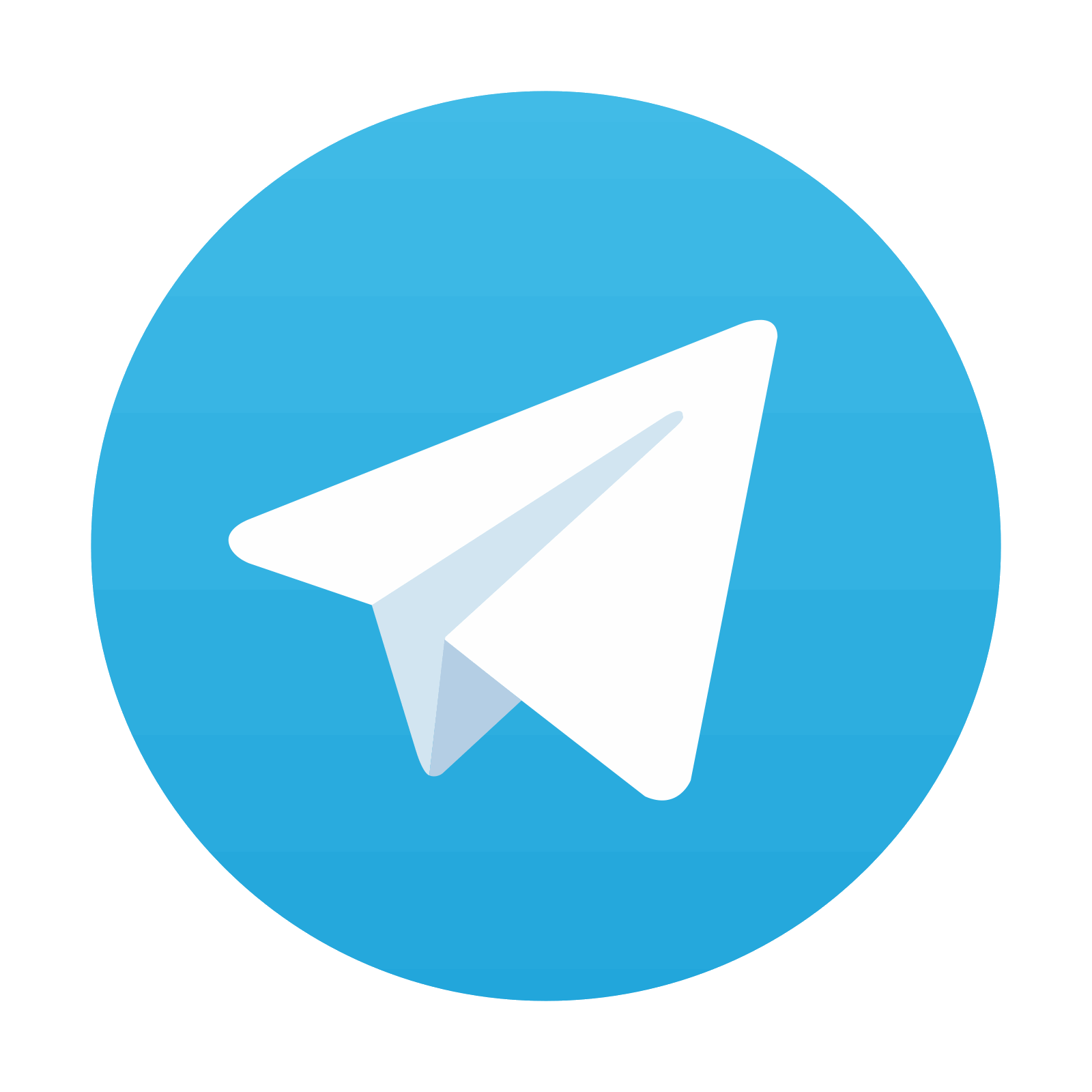
Stay updated, free dental videos. Join our Telegram channel

VIDEdental - Online dental courses
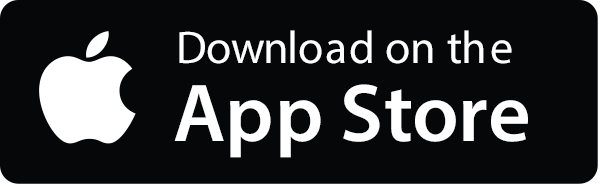
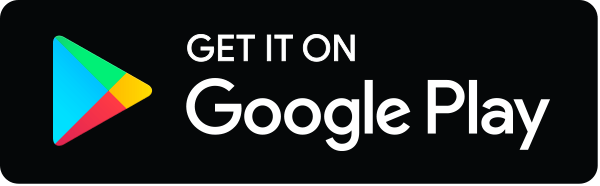