Abstract
Objective
To improve its mechanical performance, structural optimization had been used in a previous study to obtain an alternative design for a 3-unit inlay-retained fiber-reinforced composite (FRC) dental bridge. In that study, an optimized layout of the FRC substructure had been proposed to minimize stresses in the veneering composite and interfacial stresses between the composite and substructure. The current work aimed to validate in vitro the improved fracture resistance of the optimized design.
Methods
All samples for the 3-unit inlay-retained FRC dental bridge were made with glass-fibers (FibreKor) as the substructure, surrounded by a veneering composite (GC Gradia). Two different FRC substructure designs were prepared: a conventional ( n = 20) and an optimized design ( n = 21). The conventional design was a straight beam linking one proximal box to the other, while the optimized design was a curved beam following the lower outline of the pontic. All samples were loaded to 400 N on a universal test machine (MTS 810) with a loading speed of 0.2 mm/min. During loading, the force and displacement were recorded. Meanwhile, a two-channel acoustic emission (AE) system was used to monitor the development of cracks during loading.
Results
The load–displacement curves of the two groups displayed significant differences. For the conventional design, there were numerous drops in load corresponding to local damage of the sample. For the optimized design, the load curves were much smoother. Cracks were clearly visible on the surface of the conventional group only, and the directions of those cracks were perpendicular to those of the most tensile stresses. Results from the more sensitive AE measurement also showed that the optimized design had, on average, fewer cracking events: 38 versus 2969 in the conventional design.
Significance
The much lower number of AE events and smoother load–displacement curves indicated that the optimized FRC bridge design had a higher fracture resistance. It is expected that the optimized design will significantly improve the clinical performance of FRC bridges.
1
Introduction
Adhesive fixed partial denture (AFPD) was first introduced by Ibsen and Rochette in 1973. Since then, it has been recognized as a micro-invasive treatment option for caries-free dentition as, theoretically, AFPD allows the removal of healthy tooth tissues to be minimized. In the earlier designs, the framework of AFPD was mainly fabricated with a thin layer of metal alloy. With increasing patients’ demand for esthetics, ceramic materials or fiber-reinforced composite (FRC) resins started to be used for making the substructure of AFPD. However, the brittleness of ceramic materials means that larger connector dimensions and hence more tooth tissue removal are required, thus significantly limiting their clinical application . FRC bridges, on the other hand, can retain the major advantage of minimal invasion in AFPD, while providing metal-free prosthetic reconstruction with excellent esthetics. Therefore, using FRC systems to fabricate AFPD has received increasing attention from dentists and dental technicians.
However, current designs of FRC bridges do not provide an adequate lifespan. In a systematic review of FRC fixed partial dentures (FPDs), the mean survival rate was found to be 73.4% at 4.5 years . The main failure mode was documented as delamination at the interface between the fibers and the resin matrix . Fractures also occurred at the loading point , the pontic and the connectors linking the pontic to the abutments .
In addition to using stronger materials, the mechanical performance of FRC can also be improved by altering the layout of the constituent materials according to the load paths through the structure. Thus, many studies aimed to determine the optimal fiber position and orientation for the specific FRC system , the usual method being mechanical test to failure. However, the conventional load-to-failure approach has several drawbacks, for example, high material costs, time-consuming specimen preparation, and difficulty in morphological control. Furthermore, there is little knowledge of the influence of material layout on the stress distribution within the structure to guide the design process. Thus, numerical simulation such as finite element (FE) analysis has been utilized to help improve the design of FRC structures by providing a better insight into the overall stress distribution . Several FE analyses of posterior 3-unit FRC bridges indicated the presence of tensile stress concentrations at the bottom of the pontic and in the connector regions . Inadequate fiber reinforcement in these regions will lead to clinical failure.
In a recent study, an innovative shape optimization technique, the so-called stress-induced material transformation (SMT), was used in conjunction with the FE method to optimize the FRC substructure layout of a 3-unit FPD . This was achieved by gradually reinforcing the regions with high tensile stresses with FRC material such that the fibers were aligned with the direction of the maximum principal stress. Using the SMT technique, parameters such as the volume, position and orientation of the fibers in a dental restoration can be simultaneously optimized according to the stress distribution. The optimization analysis indicated a U-shape FRC substructure for the 3-unit bridge that followed the bottom profile of the pontic. The numerical results showed that the proposed optimized design significantly reduced the highest maximum principal stress in the veneering composite at both the connector and pontic positions.
The aim of this study was to validate, in vitro, the new AFPD design obtained by the SMT technique using simulated occlusal loading. In order to detect subcritical cracking more effectively, acoustic emission (AE) measurement was performed. AE is a high-frequency elastic wave produced as a result of a sudden release of strain energy within a material following, for example, a structural change such as cracking. The released strain energy causes transient sound waves that propagate through the material or structure. An AE sensor can be used to detect these sound waves so that cracking of the material or structure can be evaluated . An AE system mainly comprises three different parts: a signal sensor, a preamplifier and a recording device. AE signals collected by the AE sensors during loading are amplified with a proper gain before their amplitudes, frequencies and durations are recorded and displayed. Measuring AE signals in the mechanical testing of FRC bridges could provide real-time detection and monitoring of their fracture development with a higher sensitivity . Although AE measurement has been performed in investigations of the fracture behavior of dental materials , the present study represents the first attempt at using the AE technique for monitoring the fracture behavior of FRC bridges during in vitro mechanical testing.
2
Materials and methods
2.1
Specimen preparation
In order to prepare the FRC bridge specimens, a prosthetic restoration jaw model (D18FE-500A-QF, Nissin, Japan) was modified to duplicate the edentulous condition considered in the previous FE study . The right mandibular first molar was removed from the model and the remaining socket was filled up with paraffin wax. Then, a half-arch impression was taken for this (right mandibular) quadrant with vinyl polysiloxane impression material (Examixfine™ (injection type), GC, Japan) and silicone putty (Vertex™ Putty 1:1, Vertex-Dental, The Netherlands). Using the impression as a mold, tooth structures were recreated with a tooth-color acrylic resin (Tempron, GC, Japan) and the gingival area with a pink color acrylic resin (Palapress ® vario, Heraeus Kulzer, Germany). Two proximal boxes were prepared, respectively, in the distal region of the second premolar (with the size of 3 mm × 2 mm × 3 mm) and the mesial region of the second molar (with the size of 4 mm × 3 mm × 3 mm); see Fig. 1 a. After the proximal boxes were prepared, a second impression was taken of the duplicated right mandibular model with the same impression material mentioned above. The new mold was then poured with a stone material (Die-Keen, Columbus Dental, St. Louis, MO, USA) to fabricate the working cast for the FRC bridges.
In total, forty-one 3-unit posterior inlay FRC bridges were fabricated with unidirectional and preimpregnated S-glass fibers (FibreKor 2k bundle, Jeneric/Pentron, Wallingford, CT, USA) as the substructure, which was surrounded by a veneering composite (Gradia, GC, Japan). Fiber strips of 3 mm wide and 0.3 mm thick were used in this study. These were cut with a pair of ceramic scissors to the appropriate inter-abutment length. Three fiber strips were condensed and bonded together with a flowable composite (Filtek™ Supreme XT Flowable, 3M, USA), with each strip being light polymerized for 40 s with a curing machine (GC STEPLIGHT SL I, GC, Japan) as it was laid down in the desired position to form the FRC substructure. Two different layouts of the FRC substructure were prepared: the conventional ( n = 20) and the optimized layout ( n = 21) considered in the previous FE study . The total length of the fiber substructure, including the inter-abutment space (∼10 mm) plus the extension to the proximal boxes (∼1.5 mm on each side), was approximately 13 mm. And the thickness of the composite below the fiber substructure was approximately 3.5 mm in the conventional group and 0.5 mm in the optimized group. To ensure that differences in mechanical performance was not due to differences in fiber fraction, the same amount of glass fibers, 42 mm 3 , was used to form the FRC substructure for both groups. The conventional layout was a straight beam linking one proximal box to the other, while the optimized layout was a curved beam following the bottom profile of the pontic; see Fig. 1 b. The layer of veneering composite resin was built up incrementally and polymerized with the same curing machine (GC STEPLIGHT SL I, GC, Japan) used for curing the fibers. The morphology of the pontic mimicked the first mandibular natural molar. The occlusal surface also followed the natural profile, where the angle between buccal and lingual cusps is around 120°. The size and outline of the whole FRC bridge were controlled by a prefabricated silicone mold to ensure uniformity among the specimens. According to a previous study , using a handheld light-curing unit would not provide an adequate degree of monomer conversion and cross-linking in the resin matrix. An additional 10-min light polymerization was therefore performed with another curing unit (CURE-LITE Plus, Jeneric/Pentron, Wallingford, CT, USA) specifically built for preparing FRC bridges. Each sample was then polished with a low-speed hair brush together with a fine paste (Universal Polishing Paste, Ivoclar-Vivadent, Schaan, Lichtenstein). Before testing, all specimens were stored in de-ionized water at 37 °C for 24 h.
In addition to the two groups of FRC bridges, several resin-only specimens were made for initial trials while the experimental protocol was being refined.
2.2
Loading
A servo-hydraulic testing machine (MTS 810, MTS, Minneapolis, MN, USA) was used to apply a compressive load to the individual specimen, which was secured onto the duplicated stone model (working cast) placed at the lower part of the testing machine. The force, up to 400 N, was applied using a steel ball of 6 mm diameter and a loading speed of 0.2 mm/min on the central fossa of the pontic replacing the right mandibular first molar. The resulting deformation of the specimen could cause the bottom of the pontic to come into contact with the working cast, leading to unrepresentative loading conditions. Therefore, to prevent the above from happening, a thin layer (∼1.5 mm) of material underneath the pontic was removed from the stone cast. During loading, both the force and displacement of the loading sphere were recorded by a computer software (LJstream, LabJack, Lakewood, CO, USA) for subsequent analysis.
2.3
Acoustic emission measurement
A two-channel acoustic emission (AE) system was used to monitor the development of cracks in the specimens during loading. The AE wideband sensor (S9225, Physical Acoustic Corporation (PAC), NJ, USA) was glued onto the middle third of the buccal surface of the pontic. Signals detected by the sensor were passed through a preamplifier (Model 2/4/6, PAC, NJ, USA) with a 40 dB gain and a band pass of 100 kHz to 2 MHz. Recording of the signals was performed with the AEwin software (PAC, NJ, USA) using a sampling frequency of 1 MHz. Due to the high sensitivity of the AE sensor, a threshold value of 35 dB for the signals was imposed to filter out the background noises.
2.4
Data processing
The load–displacement data and the AE results obtained from the compressive test of each specimen were processed by using the computing software Matlab (The Mathworks, USA). As mentioned, AE signals with amplitude lower than 35 dB were ignored in order to remove the background noises. After filtering the data with the preset threshold, the accumulated number of all AE events and the amplitude of individual AE event for each specimen were extracted. Further, the sum of amplitudes from all AE events was calculated to provide a measure of the total strain energy released by each specimen. In order to relate the AE events with the load–displacement curve, the plot of AE signal amplitude versus time was superimposed upon the load–displacement curve as shown in Fig. 2 .
The first cracking event was determined from both the first abrupt drop in the load–displacement curve and the first detectable AE signal (amplitude > 35 dB). The timing and corresponding force level of the first detectable cracking event were noted from both sets of data. The mean and standard deviation of all the measured values were computed using Microsoft Excel 2007 (Microsoft, CA, USA). The T -test was used to evaluate the statistical significance of the differences between the two specimen groups: conventional and optimized design. The level of statistical significance was set at α = 0.05.
2.5
Qualitative analysis
After mechanical loading, all specimens were examined with stereo microscope (MVX10, Olympus, Japan) at 20× magnification. In order to detect subsurface cracks nondestructively, all specimens were scanned with a micro-CT system (HMX-XT 255, X-tek system, United Kingdom). Micro-CT images were taken with a tube voltage of 110 kV and a tube current of 90 μA. For each specimen, 740 projections and 64 frames per projection were acquired. The images obtained were processed using the software CT Pro (Metris, Hertfordshire, United Kingdom) with the following sequence of operation: beam hardening correction, noise reduction and three-dimensional reconstruction. After reconstruction, volume rendering of each specimen was manipulated using the software VG Studio (Version 2.0 64bit, Volume Graphics GmbH, Germany).
2
Materials and methods
2.1
Specimen preparation
In order to prepare the FRC bridge specimens, a prosthetic restoration jaw model (D18FE-500A-QF, Nissin, Japan) was modified to duplicate the edentulous condition considered in the previous FE study . The right mandibular first molar was removed from the model and the remaining socket was filled up with paraffin wax. Then, a half-arch impression was taken for this (right mandibular) quadrant with vinyl polysiloxane impression material (Examixfine™ (injection type), GC, Japan) and silicone putty (Vertex™ Putty 1:1, Vertex-Dental, The Netherlands). Using the impression as a mold, tooth structures were recreated with a tooth-color acrylic resin (Tempron, GC, Japan) and the gingival area with a pink color acrylic resin (Palapress ® vario, Heraeus Kulzer, Germany). Two proximal boxes were prepared, respectively, in the distal region of the second premolar (with the size of 3 mm × 2 mm × 3 mm) and the mesial region of the second molar (with the size of 4 mm × 3 mm × 3 mm); see Fig. 1 a. After the proximal boxes were prepared, a second impression was taken of the duplicated right mandibular model with the same impression material mentioned above. The new mold was then poured with a stone material (Die-Keen, Columbus Dental, St. Louis, MO, USA) to fabricate the working cast for the FRC bridges.
In total, forty-one 3-unit posterior inlay FRC bridges were fabricated with unidirectional and preimpregnated S-glass fibers (FibreKor 2k bundle, Jeneric/Pentron, Wallingford, CT, USA) as the substructure, which was surrounded by a veneering composite (Gradia, GC, Japan). Fiber strips of 3 mm wide and 0.3 mm thick were used in this study. These were cut with a pair of ceramic scissors to the appropriate inter-abutment length. Three fiber strips were condensed and bonded together with a flowable composite (Filtek™ Supreme XT Flowable, 3M, USA), with each strip being light polymerized for 40 s with a curing machine (GC STEPLIGHT SL I, GC, Japan) as it was laid down in the desired position to form the FRC substructure. Two different layouts of the FRC substructure were prepared: the conventional ( n = 20) and the optimized layout ( n = 21) considered in the previous FE study . The total length of the fiber substructure, including the inter-abutment space (∼10 mm) plus the extension to the proximal boxes (∼1.5 mm on each side), was approximately 13 mm. And the thickness of the composite below the fiber substructure was approximately 3.5 mm in the conventional group and 0.5 mm in the optimized group. To ensure that differences in mechanical performance was not due to differences in fiber fraction, the same amount of glass fibers, 42 mm 3 , was used to form the FRC substructure for both groups. The conventional layout was a straight beam linking one proximal box to the other, while the optimized layout was a curved beam following the bottom profile of the pontic; see Fig. 1 b. The layer of veneering composite resin was built up incrementally and polymerized with the same curing machine (GC STEPLIGHT SL I, GC, Japan) used for curing the fibers. The morphology of the pontic mimicked the first mandibular natural molar. The occlusal surface also followed the natural profile, where the angle between buccal and lingual cusps is around 120°. The size and outline of the whole FRC bridge were controlled by a prefabricated silicone mold to ensure uniformity among the specimens. According to a previous study , using a handheld light-curing unit would not provide an adequate degree of monomer conversion and cross-linking in the resin matrix. An additional 10-min light polymerization was therefore performed with another curing unit (CURE-LITE Plus, Jeneric/Pentron, Wallingford, CT, USA) specifically built for preparing FRC bridges. Each sample was then polished with a low-speed hair brush together with a fine paste (Universal Polishing Paste, Ivoclar-Vivadent, Schaan, Lichtenstein). Before testing, all specimens were stored in de-ionized water at 37 °C for 24 h.
In addition to the two groups of FRC bridges, several resin-only specimens were made for initial trials while the experimental protocol was being refined.
2.2
Loading
A servo-hydraulic testing machine (MTS 810, MTS, Minneapolis, MN, USA) was used to apply a compressive load to the individual specimen, which was secured onto the duplicated stone model (working cast) placed at the lower part of the testing machine. The force, up to 400 N, was applied using a steel ball of 6 mm diameter and a loading speed of 0.2 mm/min on the central fossa of the pontic replacing the right mandibular first molar. The resulting deformation of the specimen could cause the bottom of the pontic to come into contact with the working cast, leading to unrepresentative loading conditions. Therefore, to prevent the above from happening, a thin layer (∼1.5 mm) of material underneath the pontic was removed from the stone cast. During loading, both the force and displacement of the loading sphere were recorded by a computer software (LJstream, LabJack, Lakewood, CO, USA) for subsequent analysis.
2.3
Acoustic emission measurement
A two-channel acoustic emission (AE) system was used to monitor the development of cracks in the specimens during loading. The AE wideband sensor (S9225, Physical Acoustic Corporation (PAC), NJ, USA) was glued onto the middle third of the buccal surface of the pontic. Signals detected by the sensor were passed through a preamplifier (Model 2/4/6, PAC, NJ, USA) with a 40 dB gain and a band pass of 100 kHz to 2 MHz. Recording of the signals was performed with the AEwin software (PAC, NJ, USA) using a sampling frequency of 1 MHz. Due to the high sensitivity of the AE sensor, a threshold value of 35 dB for the signals was imposed to filter out the background noises.
2.4
Data processing
The load–displacement data and the AE results obtained from the compressive test of each specimen were processed by using the computing software Matlab (The Mathworks, USA). As mentioned, AE signals with amplitude lower than 35 dB were ignored in order to remove the background noises. After filtering the data with the preset threshold, the accumulated number of all AE events and the amplitude of individual AE event for each specimen were extracted. Further, the sum of amplitudes from all AE events was calculated to provide a measure of the total strain energy released by each specimen. In order to relate the AE events with the load–displacement curve, the plot of AE signal amplitude versus time was superimposed upon the load–displacement curve as shown in Fig. 2 .
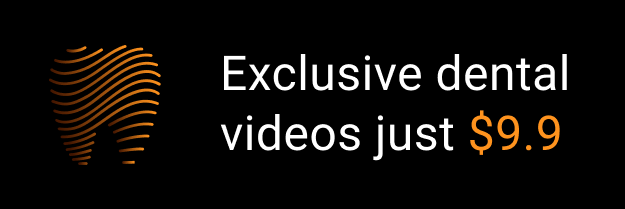