Introduction
Rapid maxillary expansion is known to improve nasal airway ventilation. However, it is difficult to precisely evaluate this improvement with conventional methods. The purpose of this longitudinal study was to use computational fluid dynamics to estimate the effect of rapid maxillary expansion.
Methods
Twenty-three subjects (9 boys, 14 girls; mean ages, 9.74 ± 1.29 years before rapid maxillary expansion and 10.87 ± 1.18 years after rapid maxillary expansion) who required rapid maxillary expansion as part of their orthodontic treatment had cone-beam computed tomography images taken before and after rapid maxillary expansion. The computed tomography data were used to reconstruct the 3-dimensional shape of the nasal cavity. Two measures of nasal airflow function (pressure and velocity) were simulated by using computational fluid dynamics.
Results
The pressure after rapid maxillary expansion (80.55 Pa) was significantly lower than before rapid maxillary expansion (147.70 Pa), and the velocity after rapid maxillary expansion (9.63 m/sec) was slower than before rapid maxillary expansion (13.46 m/sec).
Conclusions
Improvement of nasal airway ventilation by rapid maxillary expansion was detected by computational fluid dynamics.
Rapid maxillary expansion has been widely used by orthodontists to increase the maxillary transverse dimensions of young patients. Recent studies have suggested that rapid maxillary expansion also increases nasal width and volume. Therefore, rapid maxillary expansion has been generally considered to diminish the resistance of nasal airflow.
Timms reported that 82% of patients had fewer upper respiratory infections after expansion. Gray reported that, after expansion, the incidences of colds, respiratory illnesses, allergic rhinitis, and asthma were reduced by half. Therefore, rapid maxillary expansion has been suggested as a treatment option for rhinostenosis caused by septal deformity, nasal infection, allergic rhinitis, and obstructive sleep apnea. However, rapid maxillary expansion should not be encouraged as a treatment option for improvement of nasal airway ventilation conditions without an orthodontic indication.
Previous methods of evaluating nasal airway ventilation include x-rays, computed tomography, rhinomanometry, and acoustic rhinometry. However, it is difficult to take precise measurements of nasal airway ventilation with these methods because of the complicated form of the nasal airway lumen. Therefore, there is not enough evidence that nasal airway ventilation improves with rapid maxillary expansion.
To better evaluate the relationship between respiratory function and nasal morphology, a 3-dimensional (3D) model of each subject’s nasal cavity was constructed from computed tomography data and used to create computational fluid dynamics models of respiratory status during quiet respiration. Unlike some conventional methods that cannot separate nasal airflow from nasopharyngeal airflow, computational fluid dynamics can evaluate airflow in the nasal cavity alone, giving a more accurate evaluation of the effect of rapid maxillary expansion. The purpose of this study was to use computational fluid dynamics to more precisely evaluate and clarify the amount of the nasal airway ventilation improvement after rapid maxillary expansion.
Material and methods
A total of 23 patients, who visited the Kanomi Orthodontic Office (Himeji, Japan) for orthodontic treatment, participated in this longitudinal study (9 boys, 14 girls). Their mean ages before and after rapid maxillary expansion treatment were 9.74 ± 1.29 years and 10.87 ± 1.18 years, respectively. They included 10 patients with paranasal mucosa hyperplasia, 1 with adenoid tonsillar hypertrophy, and 3 with tonsillar hypertrophy. None of these subjects received surgical treatment for these conditions during the rapid maxillary expansion treatment.
All patients in this study required approximately 5 mm of maxillary expansion as part of their orthodontic treatment. Those who had previous orthodontic treatment or craniofacial or growth abnormalities were excluded. The study was reviewed and approved by the Ethics Committee of the Kagoshima University Graduate School of Medical and Dental Sciences, Kagoshima, Japan.
Each subject was seated in a chair with his or her Frankfort horizontal plane parallel to the floor. A cone-beam computed tomography scanner (CB MercuRay, Hitachi Medical, Tokyo, Japan) was set to maximum 120 kV, maximum 15 mA, and exposure time of 9.6 seconds. No subject had used a nasal decongestant at the time of the cone-beam computed tomography scan. The data were sent directly to a personal computer and stored in digital imaging and communications in medicine format.
For the evaluation of nasal and intermaxillary molar widths, a 3D coordinate system and 3D images were constructed with a medical image analyzing system (ImagnosisVE, Imagnosis, Kobe, Japan). From the 3D reconstructed images, the 3D nasal width (distance between the most lateral points of the nasal cavity) and the intermaxillary molar width (distance between the most medial points of the constricted part of the maxillary first molars) were measured to evaluate the changes produced by rapid maxillary expansion ( Figs 1 and 2 ).
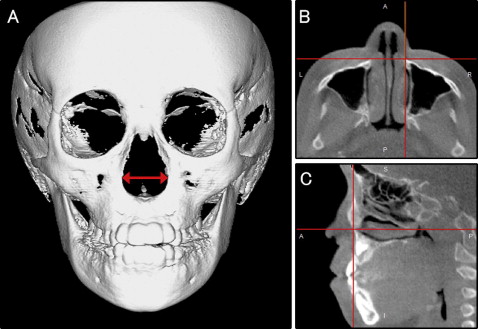
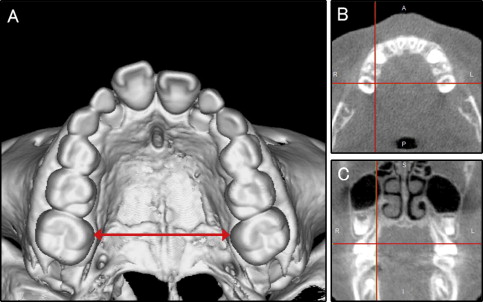
For the evaluation of the nasal airway ventilation condition, morphologic evaluation, volume-rendering software (INTAGE Volume Editor, CYBERNET, Tokyo, Japan) was used to create the 3D volume data of the nasal cavity. To construct an anatomically correct 3D model of each subject’s nasal cavity, approximately 150 slices from a computed tomography image were used. The images were obtained at intervals of 0.377 mm from the frontal sinus to the inferior nasal meatus. Because the nasal airway is a void surrounded by hard and soft tissues, inversion of the 3D-rendered image is required, converting a negative value to a positive value and vice versa. Threshold segmentation was used to select the computed tomography units in the nasal airway. Because the inverted air space has a significantly greater positive computed tomography unit than the denser surrounding soft tissue, the distinct high-contrast border produces clean segmentation of the nasal airway. By modifying the threshold limits, an appropriate range defined the tissues of interest in the volume of interest for a particular scan. By using this concept, a threshold of computed tomography units was selected to isolate all empty spaces in the nasal airway region, which also included other cavities such as the paranasal sinuses. Subsequently, by using an appropriate smoothing algorithm with a moving average, the 3D model was converted to a smooth model without losing the patient-specific character of the upper airway shape. The rendered volume data were in a 512 × 512 matrix with a voxel size of 0.377 mm. Examples of the 3D form of extracted nasal cavities (from the external nares to internal nares) and the paranasal sinuses are shown in Figure 3 . When the continuity of the bilateral nasal meatus was broken, a complete obstruction was assumed ( Fig 3 , A ).
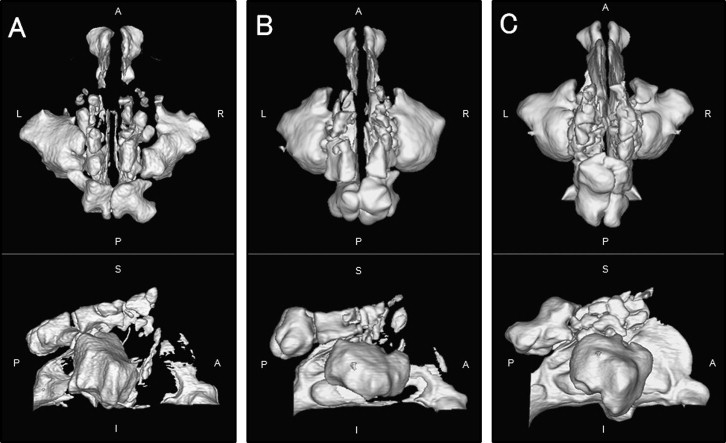
For the functional evaluation, computational fluid dynamics were used to estimate the airflow pattern of just the nasal airway ( Fig 4 ). The constructed 3D images for the nasal airway were exported to fluid-dynamic software (PHOENICS, CHAM-Japan, Tokyo, Japan) in stereolithographic format. This software can simulate and evaluate various kinds of computational fluid dynamics under a set of given conditions. In our simulation, air flowed from the choana horizontally, and air was exhaled through both nostrils. The flow was assumed to be a Newtonian, homogeneous, and incompressible fluid. Elliptic-staggered equations and the continuity equation were used in the study. The computational fluid dynamics of the nasal airway were performed under the following conditions by using PHOENICS: (1) the volume of air flowed with a velocity of 200 mL per second, (2) the wall surface was nonslip, and (3) the simulation was repeated 1000 times to calculate the mean values. Convergence was judged by monitoring the magnitude of the treatment residual sources of mass and momentum, normalized by the respective inlet fluxes. The iteration was continued until all residuals fell below 0.2%.

The simulation estimated airflow pressure and velocity. In subjects whose 3D morphologic evaluation indicated a nasal airway obstruction, computational fluid dynamics were not performed. When computational fluid dynamics indicated a maximum pressure of more than 100 Pa (with an inflow rate of 200 mL/sec) and a maximum velocity of more than 10 m per second, an obstruction was assumed in that subject.
The patients were classified into 3 groups by their ventilation conditions both before and after rapid maxillary expansion: (1) those in whom an obstruction was detected in the 3D morphologic evaluation, (2) those in whom an obstruction was detected with computational fluid dynamics but not with the 3D morphologic evaluation, and (3) those in whom no obstruction was detected with either method ( Fig 5 ).
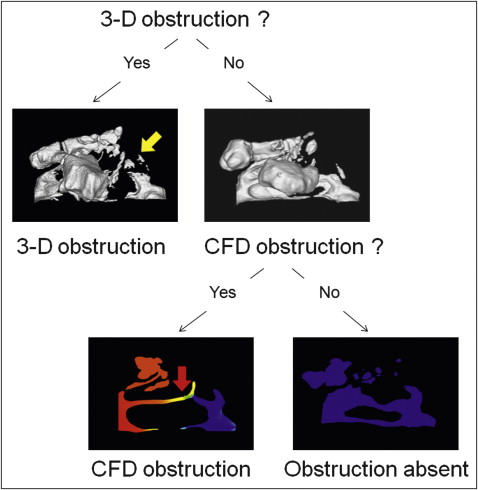
Statistical analysis
A paired t test was used to compare both the nasal and the intermaxillary molar widths before and after rapid maxillary expansion. The Mann-Whitney U test was used to compare nasal airway ventilation conditions before and after rapid maxillary expansion. Spearman correlation coefficients were calculated to evaluate the relationships between transverse dimensions and nasal airway ventilation conditions. The McNemar test was used to clarify the improvement of obstruction before and after rapid maxillary expansion. Statistical significance was set at P <0.05.
To assess the measurement error, 10 randomly selected computed tomography images from among the 46 had the nasal and intermaxillary molar widths measured twice by the same operator (T.I.) in 1 week. A paired t test detected no statistically significant differences. Dahlberg’s error of method (double determination method) was computed, and the results were 0.055 mm for nasal width and 0.072 mm for intermaxillary molar width. According to all repeated analyses, the method error was considered negligible.
Results
Nasal width after rapid maxillary expansion (23.48 ± 1.83 mm) was significantly greater than before rapid maxillary expansion (21.39 ± 1.51 mm) ( Table I ). Figure 6 shows a typical subject before and after rapid maxillary expansion. Intermaxillary molar width after rapid maxillary expansion (36.07 ± 2.74 mm) was also significantly greater than before rapid maxillary expansion (32.18 ± 2.42 mm) ( Table I ).
Before RME (n = 23) | After RME (n = 23) | Paired t test | |||
---|---|---|---|---|---|
Mean | SD | Mean | SD | P | |
Nasal width (mm) | 21.39 | 1.51 | 23.48 | 1.83 | <0.001 |
Intermaxillary molar width (mm) | 32.18 | 2.42 | 36.07 | 2.74 | <0.001 |
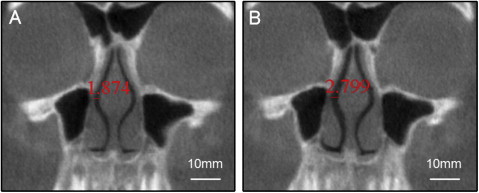
Before rapid maxillary expansion, 18 of the 23 patients (78%) had an obstruction detected by either 3D reconstruction or computational fluid dynamics. After rapid maxillary expansion, only 6 patients (26%) had a detectable obstruction ( Table II ). The change in the number of patients with obstruction after rapid maxillary expansion was statistically significant according to the McNemar test ( Table II ). A typical patient whose nasal airway ventilation improved after rapid maxillary expansion is shown in Figure 7 . Twelve of the 18 patients (66.7%) who had an obstruction before rapid maxillary expansion had no obstruction after rapid maxillary expansion.
Patient | Before RME | After RME | Ventilation improvement | Palatal expansion (mm) ∗ | ||||||
---|---|---|---|---|---|---|---|---|---|---|
Ventilation condition | Maximum pressure (Pa) | Maximum velocity (m/sec) | Ventilation condition | Maximum pressure (Pa) | Maximum velocity (m/sec) | |||||
1 | × | 3D | – | – | × | CFD | 320.8 | 29.0 | No | 2.87 |
2 | × | CFD | 131.5 | 14.0 | ○ | 9.9 | 2.2 | Yes | 4.70 | |
3 | ○ | 76.4 | 11.0 | ○ | 67.5 | 8.7 | – | 3.06 | ||
4 | ○ | 56.7 | 14.3 | ○ | 7.8 | 2.4 | – | 3.04 | ||
5 | × | CFD | 335.2 | 15.4 | ○ | 68.4 | 8.4 | Yes | 3.96 | |
6 | × | 3D | – | – | ○ | 75.5 | 9.9 | Yes | 4.04 | |
7 | × | CFD | 158.8 | 11.5 | × | CFD | 118.8 | 12.7 | No | 3.15 |
8 | × | 3D | – | – | ○ | 30.7 | 8.1 | Yes | 4.54 | |
9 | × | CFD | 117.9 | 10.4 | ○ | 35.2 | 7.1 | Yes | 5.05 | |
10 | × | CFD | 186.0 | 14.1 | × | CFD | 197.8 | 18.0 | No | 2.78 |
11 | × | CFD | 156.6 | 15.4 | ○ | 53.4 | 5.3 | Yes | 4.93 | |
12 | ○ | 25.8 | 5.4 | ○ | 27.7 | 3.7 | – | 4.81 | ||
13 | × | CFD | 327.7 | 18.8 | ○ | 64.4 | 10.7 | Yes | 5.45 | |
14 | ○ | 69.9 | 8.1 | ○ | 30.2 | 7.5 | – | 4.21 | ||
15 | × | CFD | 107.4 | 13.3 | ○ | 59.1 | 10.9 | Yes | 4.54 | |
16 | × | CFD | 106.3 | 11.4 | ○ | 63.8 | 8.1 | Yes | 3.14 | |
17 | × | CFD | 250.2 | 23.2 | × | CFD | 175.9 | 18.1 | No | 2.40 |
18 | × | CFD | 130.5 | 12.2 | ○ | 49.6 | 3.7 | Yes | 5.02 | |
19 | × | CFD | 105.1 | 12.0 | ○ | 43.6 | 3.4 | Yes | 3.52 | |
20 | × | 3D | – | – | × | 3D | – | – | No | 3.02 |
21 | ○ | 31.5 | 2.8 | ○ | 8.8 | 2.9 | – | 4.48 | ||
22 | × | 3D | – | – | ○ | 75.6 | 11.6 | Yes | 4.03 | |
23 | × | CFD | 285.2 | 29.1 | × | CFD | 187.6 | 19.8 | No | 2.69 |
Mean | 147.70 | 13.46 | 80.55 | 9.63 | 3.89 | |||||
SD | 94.87 | 5.99 | 76.59 | 6.67 | 0.91 | |||||
P | 0.008 † | 0.017 † | ||||||||
No obstruction | 5 | 17 | ||||||||
Obstruction | 18 | 6 | ||||||||
Improvement ratio | 66.7% (12/18) ‡ |
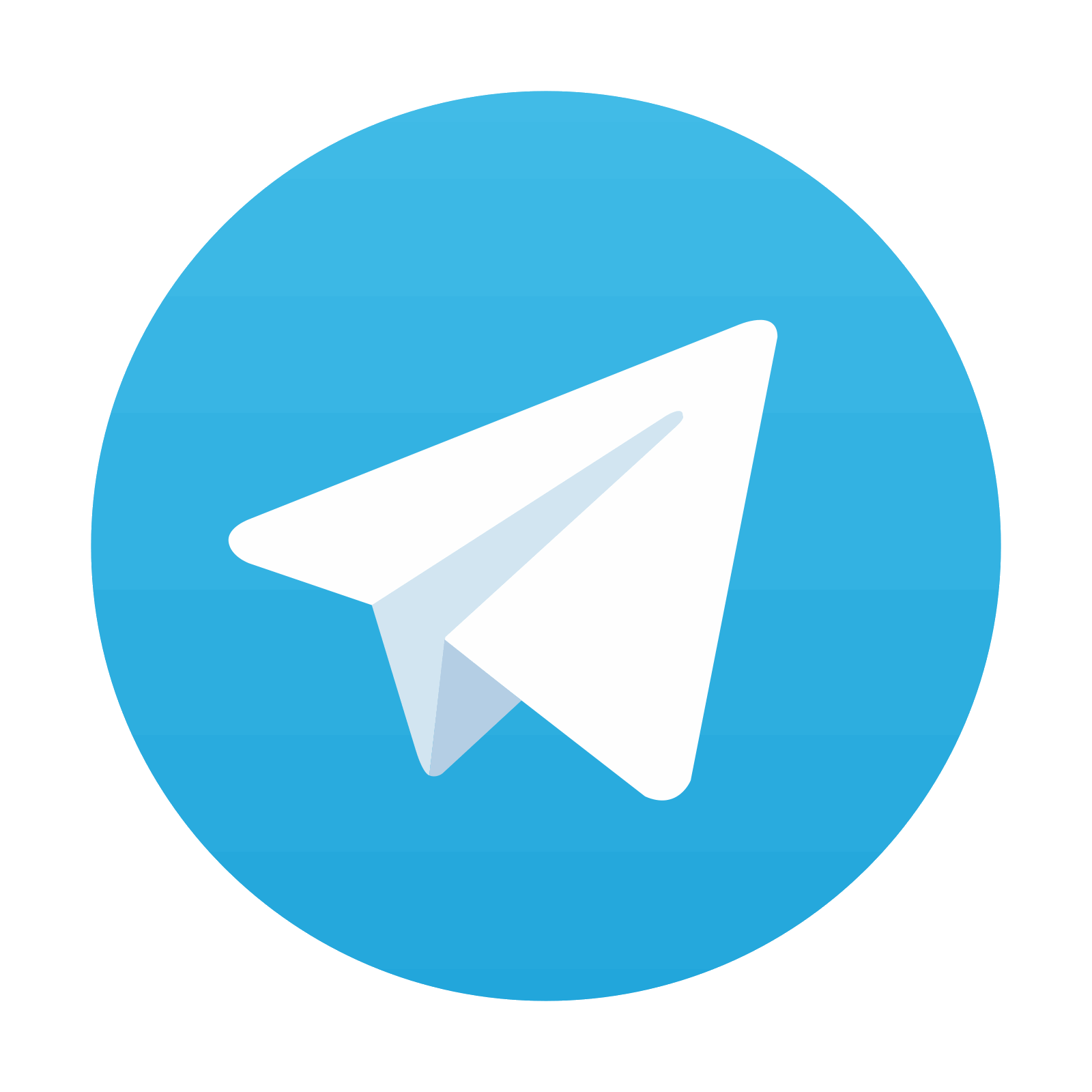
Stay updated, free dental videos. Join our Telegram channel

VIDEdental - Online dental courses
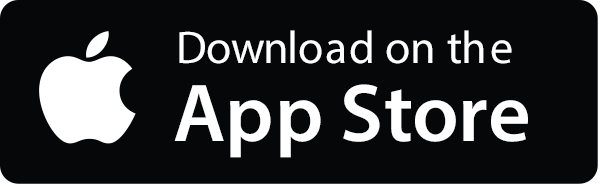
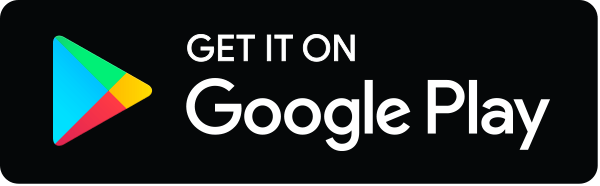
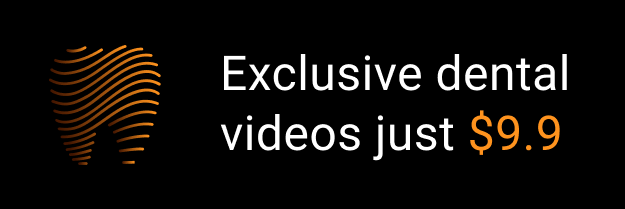