Abstract
Objectives
The bone bonding to a titanium dental implant surface strongly depends on their individual chemical surface properties. Here we hypothesize that a tailored surface chemistry obtained through a self-assembled monolayer technique on the titanium surface and subsequent immobilization of biological agents can be arbitrarily adjusted to meet any desired requirements for future dental applications.
Methods
Self-assembled monolayers were applied to titanium surfaces inducing –(CH 2 ) n CH 2 , –(CH 2 ) n –NH 2 , –(CH 2 ) n –OH, and –(CH 2 ) n –COOH functional groups. To investigate the cytocompatibility of these modifications, human mesenchymal stem cells (MSCs) were seeded on the functionalized surfaces and characterized by live/dead staining and cell proliferation. Additionally, bovine serum albumin (BSA) as a model protein was immobilized on the functionalized surfaces.
Results
Water contact angle measurements and X-ray photoelectron spectroscopy (XPS) proved a successful functional group coupling on the surface. Cell proliferation and viability was supported on the functionalized surfaces. The immobilized proteins were detected on each functionalized surface with an increase in quantity in the following order: –(CH 2 ) n –COOH< –(CH 2 ) n –NH 2 , –(CH 2 ) n –OH< –(CH 2 ) n CH 2 . The successful immobilization of BSA by carboxyl-to-amine cross-linking was spectrophotometrically confirmed.
Significance
It was proved that self-assembled monolayer (SAM)-technique can be utilized to couple diverse functional groups and biological agents on titanium surfaces allowing controlled design of their surface chemistry. The novel functionalization technique and the new knowledge on MSCs response holds great potential for the development of novel functionalized and biologically activated titanium-based dental implants.
1
Introduction
Titanium (Ti) possesses good mechanical properties as well as surface properties capable of eliciting biological responses, making it a versatile and commonly used surgical implant material especially in dentistry . Upon exposure of Ti to oxygen, native oxides emerge on the surface . Due to the fact that this titanium oxide is basically bioinert , various methods have been developed to improve the bone bonding capability to the titanium implant surface. The majority of the investigated modifications so far are intended to accelerate osseointegration in order to increase the implants ability to bond to the surrounding tissue and ultimately decrease the risk of implant failure. Such techniques include e.g. deposition of sol–gel derived calcium phosphate or application of crystalline hydroxyapatite coating . However, some studies came to the conclusion that thin CaP-based coatings could degrade under in vivo conditions, causing the implants to loosen . An additional commonly occurring disadvantage of this functionalization method is mechanical failure at the interface between the CaP-layer and the underlying titanium substrates .
An alternative method to bioactive coatings constitutes a chemical modification of the surface using a self-assembled monolayer (SAM) technique. SAMs can be formed by introducing silane coupling agents to hydroxylated surfaces . Here, a polymerization process causes the respective surface to be effectively coated with a polymer–monolayer of the silane monomer precursor, forming a variety of subsequent surface modification possibilities. A huge advantage of an individually tailored SAM surface is the ability to create well-ordered organic surfaces that provide control over the properties of the interface at the molecular scale . This interface can then be further modified by the introduction of different functional end groups. Various studies have shown the successful coupling of SAMs on Ti-based-surfaces altered by several functional end groups (–OH, –COOH, –NH 2 , –PO 4 H 2 , –CH CH 2 , –CH 3 ) for hydroxyapatite deposition . Liu et al. developed –CH CH 2 , –OH, –PO 4 H 2 , and –COOH surface functional groups on Ti-foils and investigated their effect on calcium phosphate nucleation. However, success was reported only on oxidized Ti and subsequently carboxylated SAM . Liu et al. applied –OH– and –COOH-functional groups to Ti specimens and were able to show that –COOH functional groups provide an optimal SAM interface for calcium phosphate nucleation purposes .
Additionally SAMs have been found useful to investigate the effect of surface chemistry on various proteins and cells . It is well known, that upon contact with blood–plasma or –serum, which are physiological protein-rich media, surfaces get immediately covered with layers of adsorbed proteins, and that the resulting cell response is largely mediated by the nature and bioactivity of those proteins in a highly complex and dynamic process . Therefore, various studies investigated the immobilization process of proteins on individually modified Ti surfaces . One of the common methods for immobilization of a biological agent to the functionalized Ti-surfaces is the cross-linking technique , which is defined as the process of chemically joining two or more monomers by a covalent bond.
Depending on the desired application it is possible to select the specific protein for a targeted influence on the cell response. Puleo et al. investigated BMP-4 bound to Ti–Al6–V4, expressing a high density of amino groups for improvement of cell osseointegration. Here, the protein absorption was carried out using two different schemes. In a one-step scheme the protein was coupled with aminated surfaces using the crosslinking agents EDAC and NHS. In a two-step scheme, the protein was coupled by EDAC and NHS following a previous conversion of the amino groups to carboxyl groups . Both approaches showed successful protein immobilization on the activated Ti-surface. However, this study has also shown that the crosslinking of proteins can confine their activity potential. Li et al. applied the described functionalization techniques for implants with blood cell contact to improve their hemocompatibility and to avoid thrombosis formation . Here, a co-immobilization of heparin and fibronectin was successfully implemented on the amino-silanized titanium surfaces. Subsequent endothelial cell culture tests have proven that the co-immobilization of these two proteins potentially improves the hemocompatibility and stimulates endothelialization.
Another possible application of protein immobilization using the SAM-technique is to diminish bacterial infection, which is among the most frequent reasons of implant failure in dentistry . It was proven, that the functionalization of titanium surfaces with the hLf1-11 peptide using silanization reduced the adhesion and early stages of biofilm formation, and inhibited the early stage of bacterial growth.
The listed examples were chosen to display the great intrinsic potential of chemical modification and biologization of titanium surfaces using the SAM-technique for dental applications. Nevertheless, the majority of conducted studies suggest protein immobilization using aminated surfaces, which requires the direct cross-linking of proteins. Other studies however have proven that through the cross-linking of proteins their activity and consequently their function becomes weaker or vanishes completely . In the present study, we therefore demonstrate the immobilization of biological agents using the example of bovine serum albumin (BSA) to four functional surface groups on titanium (–(CH 2 ) n –CH 2 , –(CH 2 ) n –OH, –(CH 2 ) n –COOH, and –(CH 2 ) n –NH 2 ) by different immobilization methods. We hypothesize, that using a self-assembled monolayer technique, a tailored chemistry of the titanium surface and subsequent immobilization of biological agents can be arbitrarily adjusted to meet any desired requirements. Furthermore, we investigate the influence of the various chemical pretreatments of Ti with different surface functional groups on the coupling of BSA without an additional cross-linking agent.
2
Materials and methods
2.1
Preparation of Ti surfaces
Titanium specimens were prepared from a 99.7% pure Ti sheet (thickness: 2 mm, Aldrich, Steinheim, Germany) which was cut into circular samples with diameters of either 6 mm or 12 mm and subsequently polished to a final surface roughness of R a = 0.15 μm. The polished specimens were successively rinsed with distilled water and acetone (Sigma–Aldrich Chemie, Steinheim, Germany) and air-dried under atmospheric conditions. Surface oxidization was achieved by immersing the samples in “piranha” solution (1:1 (v/v) mixture of concentrated H 2 SO 4 and 30% H 2 O 2 (Sigma–Aldrich Chemie, Steinheim, Germany)) for 1 h at ambient temperature, following the method previously described by Liu et al. . Lastly, the specimens were washed again three times in distilled water and two times in acetone and air-dried at ambient temperature followed by oven-drying at 80 °C for 15 min.
2.2
Self-assembled monolayers preparation
The self-assembled monolayers (SAMs) were prepared on the hydroxylated titanium surfaces to achieve four different functional end groups: –(CH 2 ) n CH 2 , –(CH 2 ) n –OH, –(CH 2 ) n –COOH, and –(CH 2 ) n –NH 2 . To create a monolayer with –(CH 2 ) n –CH 2 functional groups octyltrichlorosilane (OCTS trichloro(octyl)silane, mixture of isomers 97%, Sigma–Aldrich Chemie, Steinheim, Germany) was used. The specimens were immersed in 20 ml of 5% OCTS-solution for 1 h at room temperature, whereby anhydrous ethanol was used as solvent (ethanol anhydrous <0.0030% H 2 O, VWR International, Darmstadt, Germany). Afterwards the specimens were rinsed three times in anhydrous ethanol and were air-dried.
To create specimens with –(CH 2 ) n –OH and –(CH 2 ) n –COOH functional end groups, samples already containing the –(CH 2 ) n CH 2 functional group were used. The coupling of hydroxyl groups was achieved by hydroboration followed by an oxidation process. For hydroboration the OTS-treated specimens were immersed into 10 ml of 1 M B 2 H 6 /THF (VWR International, Darmstadt, Germany) complex solution for 30 min at ambient temperature. Hydroxylation took place after the specimens were transferred into a solution of 0.1 M NaOH and 30% H 2 O 2 (Sigma–Aldrich Chemie, Steinheim, Germany) for 5 min. Afterwards, the specimens were washed with distilled water and air-dried. For carboxylation the specimens were immersed in 10 ml of 5% acidic KMnO 4 for 5 min at ambient temperature. The specimens were thoroughly rinsed with distilled water and air-dried.
The –(CH 2 ) n –NH 2 terminated monolayer was created by using 10 ml of a 5% solution of 3-aminopropyltriethoxysilane (APTS, 3-aminopropyltriethoxysilane, 99%, Sigma–Aldrich Chemie, Steinheim, Germany) in anhydrous toluol (toluol anhydrous < 0.0030% H 2 O, VWR International, Darmstadt, Germany) in which the oxidized titanium specimens were immersed and allowed to react in a reflux condenser at 120 °C for 3 h. Afterwards the room-temperature specimens were rinsed three times in anhydrous toluol, followed by three times washing in distilled water and air drying.
2.3
Water contact angle measurement
Static water contact angle measurement on the prepared specimens was performed at ambient temperature. To determine the influence of the various treatments on the surface-hydrophobicity and -charge a single droplet of distilled water was depositioned on the modified surfaces for each measurement and subsequently imaged using a compact video microscope (Compact Videomicroscope, PW Allen, Tewkesbury, UK). The droplet-surface contact angles were analyzed using a contact angle plugin in the image processing software ImageJ . Each specimen was subject to three independent measurements evenly distributed on its surface.
2.4
Chemical analysis
X-ray photoelectron spectroscopy (XPS Ultra Axis spectrometer, Kratos Analytical, Manchester, UK) was performed to characterize the elemental surface composition after each modification. The chemical composition of each specimen, given in percent, was taken from the survey spectra.
2.5
Protein immobilization
For the protein immobilization studies bovine serum albumin (BSA, 98% purity, Sigma–Aldrich Chemie, Steinheim, Germany) was used in high concentrations (10 mg/ml) to provide an excess of protein in solution available to react with the activated functional groups on the Ti surfaces. For –(CH 2 ) n –NH 2 and –(CH 2 ) n –COOH functionalized surfaces a cross-linking technique was employed. Here, BSA was linked by carboxyl-to-amine cross-linking using the carbodiimide1-ethyl-3-(-3-dimethylaminepropyl) (EDC) (1-ethyl-3-(-3-dimethylaminepropyl), Thermo Fisher Scientific, Rockford, USA) and N-hydroxysuccinimide (NHS) (N-hydroxysuccinimide, Thermo Fisher Scientific, Rockford, USA). Fig. 1 shows EDC and NHS reaction chemistry. Briefly, EDC reacts with carboxylic acid groups to form an active ester intermediate which in turn is easily displaced by nucleophilic attack from primary amino groups in the reaction mixture. The primary amine forms an amide bond with the original carboxyl group and an EDC by-product is released as a soluble urea derivative. NHS esters are reactive groups formed by carbodiimide-activation of carboxylate molecules. NHS ester-activated cross-linkers and labeling compounds react with primary amines to yield stable amide bonds.

2.5.1
Cross-linking of proteins
To achieve protein immobilization on specimens with –CH 2 –NH 2 functional end groups 500 mg BSA was solved in 50 ml 2-morpholinoehtane sulfonic acid (MES, pH 5.5, Thermo Fisher Scientific, Rockford, USA) and mixed with 20 mg EDC and 30 mg NHS. The resulting solution was allowed to react for 15 min at room temperature after which 10 μl 2-mercaptoethanol (Aldrich Chemie, Steinheim, Germany) was added to the solution to inactivate the EDC component. Each specimen was submerged in 500 μl of the prepared protein solution with an addition of 500 μl phosphate buffered saline (PBS, pH 7.4, Thermo Fisher Scientific, Rockford, USA) and allowed to react for 2 h at ambient temperature. Afterwards the specimens were rinsed three times in PBS and two times in distilled water.
2.5.2
Cross-linking of surface functional groups
To achieve protein immobilization on specimens with –(CH 2 ) n –COOH functional end groups 20 mg EDC and 30 mg NHS were mixed with 50 ml MES. 1 ml of the resulting solution was transferred to each modified sample where it reacted for 15 min at ambient temperature. The specimens were subsequently rinsed once with PBS. Afterwards 1 ml of the 10 mg/ml BSA-solution described above was given to each specimen. After a reaction time of 2 h at ambient temperature, the specimens were rinsed three times in PBS and two times in distilled water.
2.5.3
Spontaneous chemisorption
To investigate spontaneous protein immobilization on specimens with –(CH 2 ) n –CH 2 and –(CH 2 ) n –OH functional end groups 1 ml of the 10 mg/ml BSA-solution without cross-linking agents was transferred to each specimen with a subsequent reaction time of 2 h at ambient temperature. The specimens were afterwards rinsed three times in PBS and two times in distilled water.
For control purposes, untreated oxidized titanium specimen without any further surface modification were subjected to each of the protein immobilization methods as described above, including all subsequent steps, respectively.
2.6
Histochemistry
Histochemistry was prepared on two consecutive days. In a first step 5% paraformaldehyde (PAF, Merck, Darmstadt, Germany) was pipetted on each specimen and allowed to react for 15 min. Afterwards the specimens were rinsed three times in PBS. Then 2% horse serum blocking solution (Donor Horse Serum, Biowest SAS, Nuaillé, France) was pipetted to each specimen for a reaction time of 1 h. Subsequently primary anti-BSA antibody (policlonal anti-bovine serum albumin antibody produced in rabbit, antibodies-online, Aachen, Germany) was used in a 1:200 dilution. The specimens were incubated overnight. On the next day the antibody solution was aspirated and the specimens were rinsed three times in PBS. The second antibody, Alexa Fluor 488 Anti-Rabbit IgG was applied in the concentration of 1:200 as well and incubated for 2.5 h. After incubation the antibody solution was aspirated and the specimens were rinsed three times in PBS.
2.7
Protein detection
For quantification of the total adsorbed protein content a Pierce BCA Protein Assay Kit (Pierce Biotechnology, Rockford, USA) was used. The extract was analyzed in a spectrophotometer (Tecan Reader Infinite, Tecan Group, Männedorf, Switzerland) at 562 nm and compared to a standard dilution as previously described.
2.8
Cell culture
Mesenchymal stem cells (MSCs) were prepared from human bone marrow aspirate. The MSCs were seeded in 24-well-plates at a density of 5000 cells/cm 2 for proliferation and life/dead staining experiments. The cells were cultured in Mesenpan MSC medium (PAN-Biotech, Aidenbach, Germany) and incubated at 37 °C in a 20% O 2 and 5% CO 2 humidified atmosphere. Medium was changed every three to four days.
2.8.1
Live/dead staining
Live/dead staining was performed after four days of incubation as described previously . The staining was carried out on pure (Ti), hydroxylated titanium (Ti–OH), titanium with the functional groups (–(CH 2 ) n –CH 2 , –(CH 2 ) n –OH, –(CH 2 ) n –COOH, –(CH 2 ) n –NH 2 ) and glass (positive control).
2.8.2
Cell proliferation
At one, four, and seven days after cell seeding CellTiter-Blue cell viability test (Promega, Mannheim, Germany) was used to quantify proliferating cells. The test was performed on the same type of specimen as the live/dead staining but with TCPS (Tissue Culture Polystyrene) as positive control.
2.8.3
Statistics
Statistical significance was calculated by the unpaired Student’s t -test with an error probability p . Differences were considered as statistically significant for p < 0.05.
2
Materials and methods
2.1
Preparation of Ti surfaces
Titanium specimens were prepared from a 99.7% pure Ti sheet (thickness: 2 mm, Aldrich, Steinheim, Germany) which was cut into circular samples with diameters of either 6 mm or 12 mm and subsequently polished to a final surface roughness of R a = 0.15 μm. The polished specimens were successively rinsed with distilled water and acetone (Sigma–Aldrich Chemie, Steinheim, Germany) and air-dried under atmospheric conditions. Surface oxidization was achieved by immersing the samples in “piranha” solution (1:1 (v/v) mixture of concentrated H 2 SO 4 and 30% H 2 O 2 (Sigma–Aldrich Chemie, Steinheim, Germany)) for 1 h at ambient temperature, following the method previously described by Liu et al. . Lastly, the specimens were washed again three times in distilled water and two times in acetone and air-dried at ambient temperature followed by oven-drying at 80 °C for 15 min.
2.2
Self-assembled monolayers preparation
The self-assembled monolayers (SAMs) were prepared on the hydroxylated titanium surfaces to achieve four different functional end groups: –(CH 2 ) n CH 2 , –(CH 2 ) n –OH, –(CH 2 ) n –COOH, and –(CH 2 ) n –NH 2 . To create a monolayer with –(CH 2 ) n –CH 2 functional groups octyltrichlorosilane (OCTS trichloro(octyl)silane, mixture of isomers 97%, Sigma–Aldrich Chemie, Steinheim, Germany) was used. The specimens were immersed in 20 ml of 5% OCTS-solution for 1 h at room temperature, whereby anhydrous ethanol was used as solvent (ethanol anhydrous <0.0030% H 2 O, VWR International, Darmstadt, Germany). Afterwards the specimens were rinsed three times in anhydrous ethanol and were air-dried.
To create specimens with –(CH 2 ) n –OH and –(CH 2 ) n –COOH functional end groups, samples already containing the –(CH 2 ) n CH 2 functional group were used. The coupling of hydroxyl groups was achieved by hydroboration followed by an oxidation process. For hydroboration the OTS-treated specimens were immersed into 10 ml of 1 M B 2 H 6 /THF (VWR International, Darmstadt, Germany) complex solution for 30 min at ambient temperature. Hydroxylation took place after the specimens were transferred into a solution of 0.1 M NaOH and 30% H 2 O 2 (Sigma–Aldrich Chemie, Steinheim, Germany) for 5 min. Afterwards, the specimens were washed with distilled water and air-dried. For carboxylation the specimens were immersed in 10 ml of 5% acidic KMnO 4 for 5 min at ambient temperature. The specimens were thoroughly rinsed with distilled water and air-dried.
The –(CH 2 ) n –NH 2 terminated monolayer was created by using 10 ml of a 5% solution of 3-aminopropyltriethoxysilane (APTS, 3-aminopropyltriethoxysilane, 99%, Sigma–Aldrich Chemie, Steinheim, Germany) in anhydrous toluol (toluol anhydrous < 0.0030% H 2 O, VWR International, Darmstadt, Germany) in which the oxidized titanium specimens were immersed and allowed to react in a reflux condenser at 120 °C for 3 h. Afterwards the room-temperature specimens were rinsed three times in anhydrous toluol, followed by three times washing in distilled water and air drying.
2.3
Water contact angle measurement
Static water contact angle measurement on the prepared specimens was performed at ambient temperature. To determine the influence of the various treatments on the surface-hydrophobicity and -charge a single droplet of distilled water was depositioned on the modified surfaces for each measurement and subsequently imaged using a compact video microscope (Compact Videomicroscope, PW Allen, Tewkesbury, UK). The droplet-surface contact angles were analyzed using a contact angle plugin in the image processing software ImageJ . Each specimen was subject to three independent measurements evenly distributed on its surface.
2.4
Chemical analysis
X-ray photoelectron spectroscopy (XPS Ultra Axis spectrometer, Kratos Analytical, Manchester, UK) was performed to characterize the elemental surface composition after each modification. The chemical composition of each specimen, given in percent, was taken from the survey spectra.
2.5
Protein immobilization
For the protein immobilization studies bovine serum albumin (BSA, 98% purity, Sigma–Aldrich Chemie, Steinheim, Germany) was used in high concentrations (10 mg/ml) to provide an excess of protein in solution available to react with the activated functional groups on the Ti surfaces. For –(CH 2 ) n –NH 2 and –(CH 2 ) n –COOH functionalized surfaces a cross-linking technique was employed. Here, BSA was linked by carboxyl-to-amine cross-linking using the carbodiimide1-ethyl-3-(-3-dimethylaminepropyl) (EDC) (1-ethyl-3-(-3-dimethylaminepropyl), Thermo Fisher Scientific, Rockford, USA) and N-hydroxysuccinimide (NHS) (N-hydroxysuccinimide, Thermo Fisher Scientific, Rockford, USA). Fig. 1 shows EDC and NHS reaction chemistry. Briefly, EDC reacts with carboxylic acid groups to form an active ester intermediate which in turn is easily displaced by nucleophilic attack from primary amino groups in the reaction mixture. The primary amine forms an amide bond with the original carboxyl group and an EDC by-product is released as a soluble urea derivative. NHS esters are reactive groups formed by carbodiimide-activation of carboxylate molecules. NHS ester-activated cross-linkers and labeling compounds react with primary amines to yield stable amide bonds.
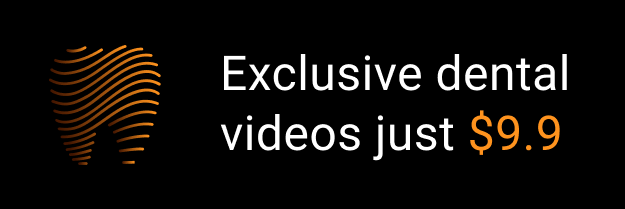