14
Hydraulic Calcium Silicate-based Endodontic Cements
Josette Camilleri
Summary
Hydraulic calcium silicate cements were introduced to dentistry initially for perforation repair and to be used as root-end filling materials. The use of such materials, which originated from the construction industry, had the specific purpose of usage in wet environments such as surgical sites. Since their introduction in the mid-1990s, there have been several exciting developments in these materials and this group of hydraulic cements have now been recognised to have a large number of uses in endodontic practice.
This chapter covers the properties and composition of these materials and goes into detail about the cement, radiopacifier, additive, and vehicle characteristics and the implications of these in clinical use. The material properties are closely related to cement characteristics and the interaction of the cement with tooth structure and blood and tissue fluids. The radiopacifier, additives, and the vehicle modify the cement hydration process and the physical and biological properties.
A classification for these materials is proposed based on the material chemistry that will help clinicians to understand the differences in the types of material as this has clinical implications. The properties related to how each specific material should be used is discussed. Their use is subdivided into coronal and radicular. The intracoronal use includes materials used for pulp preservation, and those used for the management of both vital and nonvital pulps in immature teeth. The radicular use incorporates the intraradicular use of materials including the root canal sealers, apical plugs in immature teeth with nonvital pulps, and materials used to repair root perforations nonsurgically and their extraradicular use as root-end filling materials and materials used for surgical repair of root perforations. The clinical requirement for each of these materials varies.
The current challenges within this field include developing a consensus on proper nomenclature and the understanding of the interactions of specific materials is discussed together with the need for proper clinical protocols. The interactions of these materials limit their proper use unless specific protocols based on material chemistry are adopted. The need for standardisation and the creation of appropriate standards for laboratory research is also highlighted.
14.1 Introduction
Hydraulic calcium silicate-based cements have a variety of uses in clinical endodontics [1]. Initially in the mid-1990s, they were specifically developed for perforation repair [2] and root-end filling [3]. The idea of developing these materials was based on the hydraulic nature of Portland cement used in the construction industry. Portland cement develops its physical characteristics when wet. Because root-end filling and perforation repair materials have to be placed in wet environments, Mahmoud Torabinejad and colleagues translated the use of Portland cement for use in clinical dentistry. Although his invention, which is patented [4, 5], has been developed considerably, it was not the first time that Portland cement has been used in dentistry.
The first scientific publication of the use of Portland cement in dentistry can be attributed to Witte in 1878 [6]. The first patented formulation containing Portland cement was called mineral trioxide aggregate (MTA). Although the patents [4, 5] clearly identify the composition as Portland cement, even with a suggested trade name and bismuth oxide added to enhance the radiopacity, the first publication identifying the chemical composition of MTA indicated the presence of two phases: calcium oxide and calcium phosphate. Further analysis demonstrated that the former appeared as discrete crystals and the latter as an amorphous structure with no apparent crystal growth but a granular appearance. The mean value of the prisms was 87% calcium and 2.47% silica, the remainder being oxygen. In areas of amorphous structure, there seemed to be 33% calcium, 49% phosphate, 2% carbon, 3% chloride, and 6% silica [7]. This was a common belief for nearly a decade until it was discredited in later material characterisation work [8–11]. It was shown that MTA was composed of Portland cement, which is made up of tricalcium and dicalcium silicate, tricalcium aluminate and tetracalcium aluminoferrite, and bismuth oxide to act as a radiopacifier [8]. Due to the fact that the material was shown to be a silicate, the name mineral trioxide aggregate would appear to be inappropriate. The presence of phosphate was due to material contamination during preparation for scanning electron microscopy (SEM) [12] as MTA is phosphate-free.
The first MTA was manufactured by Dentsply Sirona (Tulsa, OK, USA) and was marketed as ProRoot. There was very little development in this field due to the block of any further material development by the U.S. patent applications [4, 5]. Later, Dentsply Sirona developed a white MTA [13, 14]. This development was believed to be the solution to tooth discoloration reported with grey MTA [15]. The only other development was the introduction of MTA Angelus (Angelus, Londrina, Brazil). This material was limited for sale in Brazil initially until 2005 after which more materials were developed and marketed for clinical use.
14.2 Material Properties
The properties of tricalcium silicates for endodontic use involve mostly the specific chemistry of cement, but the radiopacifier used also plays a role in the characteristics of the material and the further development of these material types. There are various types of hydraulic cements in clinical use and their composition is summarised in Figure 14.1. All of them have a cement component that is based on tricalcium or dicalcium silicate cement. Most materials have a radiopacifier to enable radiographic detection postoperatively. The classical cements are mixed with water to enable hydration, but modified cements can be premixed or light curable. A number of additives are used to enhance material properties.

Figure 14.1 Diagrammatic representation of hydraulic cements in clinical use showing the main components of the different material types.
14.2.1 Cement Characteristics
The main component of MTA is tricalcium silicate [16]. When tricalcium silicate is mixed with water, it forms calcium silicate hydrate and calcium hydroxide. This has been well recognised in the civil engineering field for industrial Portland cement [17–19] and for MTA [9, 10].
The tricalcium silicate hydration reaction is shown in Equation 14.1. In Portland cement, due to the presence of other phases such as dicalcium silicate and tricalcium aluminate, further hydration reactions occur as shown in Equations 14.2 and 14.3.



The end product of hydration of tricalcium silicate is calcium silicate hydrate and calcium hydroxide whereas that of MTA also includes ettringite and monosulphate; the proportions depend on the amount of free sulphate available from the gypsum during the reaction.
Calcium hydroxide is used extensively in Dentistry. Its uses include pulp related procedures, as a dressing in the root canal in between root canal treatment visits, and as a root canal sealer. Calcium hydroxide has both antimicrobial and biological properties. The specific properties are discussed later with each clinical application.
The main features of tricalcium silicate-based cements is the hydraulic nature that makes the material suitable for use in aqueous and humid environments. Furthermore, the formation of calcium hydroxide provides additional uses for the material. The calcium and hydroxide ions in solution interact with the clinical environment and lead to specific phenomena. This happens for all material types if the hydration reaction occurs. Hydraulic cements are used for pulp preservation procedures where they are in contact with coronal dentine and blood and tissue fluids; as barrier materials in regenerative endodontic procedures in contact with a blood clot; as apical plugs in immature teeth in contact with radicular dentine; as root canal sealers also in contact with radicular dentine; for root-end filling, in contact with radicular dentine and blood; and for perforation repair where, depending on the site of the perforation, the material is in contact with dentine, other restorative materials, and blood.
14.2.1.1 Hydraulic Cement Interaction with Dentine
Hydraulic cements exhibit specific interactions in clinical use. The interaction with coronal dentine has been studied using various microscopy techniques. The ionic exchange across the interface has been reported using confocal microscopy. With confocal microscopy, a fluorescent dye is added to the material and the movement of the dye is mapped by the confocal microscope. A mineral infiltration zone forms in dentine in contact with the hydraulic cement (Figure 14.2a). This zone forms by alkaline etching, which facilitates the movement of ions across the interface [20]. In the same study using SEM, an altered material microstructure was observed at the tooth to material interface (Figure 14.2b). Another study using SEM and elemental line scans accompanied by Raman spectroscopy showed the deposition of phosphorus on the material surface at the interface [21]. This was confirmed by another study where amorphous calcium phosphate was formed at the interface of hydraulic cement with coronal dentine [22].

Figure 14.2a Representative fluorescence-mode CLSM images for the interfaces of Biodentine and GIC cements (upper side of each image) with the dentine (lower side). (a1) Distribution of the fluorescein dye labeling the Biodentine cement shows a band of richly dye-infiltrated dentine just beneath the interface. This band cannot be seen with Rhodamine-B micro-permeability, (b1) where the dye permeated through this band and diffused into the cement above the interface without any mixing with the fluorescein. This band is believed to be formed as a result of the strong alkaline effect of calcium hydroxide leaching out of the cement, and it corresponds to the MIZ. (c1) Dye-deficient areas can be seen within this zone (arrows), which represent the unaffected peritubular dentine. (a2) Fluorescein distribution in GIC samples also shows a richly dye-infiltrated dentine band, but this band was formed due to the acidic effect of GIC, which affected both intertubular and peritubular dentine. (b2) Rhodamine-B permeating from the pulp has also infiltrated this band, which diffused laterally through the affected tubular walls and mixed with the fluorescein as shown in (c2). (d1) Second-harmonic generation (SHG) signal, originating from the intertubular collagen (cyan), is weak and almost absent in the interfacial dentine beneath the Biodentine (arrows) when superimposed over the dentine’s autofluorescence signal (red), which reflects its actual margin. Notice the preserved tubular structure of dentine in this zone, unlike the images for GIC samples (d2), where the SHG signal has the same distribution as the dentine’s autofluorescence signal. (Reproduced from Atmeh et al. [20] / with permission from SAGE Publications).

Figure 14.2b SEM micrographs of the fractured dentine discs, which were exposed to (a) Biodentine (b) or GIC. A band of structurally altered dentine extends along the interface, as can be seen by the obliterated dentine tubules above the dashed line in (a), when compared with dentine of the opposite surface of the disc, where no cement was applied (c). (b) No structural changes can be detected in the dentine beneath GIC when compared with dentine of the opposite surface (d). (Reproduced from Atmeh et al. [20] / with permission from SAGE Publications.)
The interaction of hydraulic sealers with radicular dentine also shows the mineral infiltration zone when confocal microscopy is used to assess the tooth to material interface (Figure 14.3a) and elemental migration was also shown [23, 24], which was mostly conspicuous for silicon and phosphorus (Figure 14.3b). Calcium cannot be mapped unless the calcium in the material is tagged with a calcium binding dye such as Fluo 3 [25] that enables the differentiation of the calcium in the material form of the tooth. In hydraulic cements containing zirconium, the zirconium and phosphorus show overlapping peaks so the migration of these elements cannot be mapped separately [23].

Figure 14.3a Confocal micrographs showing the interface of root canal sealers in contact with root dentine showing their interaction with dentine and sealer penetration in the dentinal tubules. (Reproduced from Kebudi Benezra et al. [24] / with permission from Elsevier).

Figure 14.3b Scanning electron micrographs and elemental maps of various elements showing elemental distribution in the material matrix of BioRoot RCS and energy dispersive spectroscopic maps of the tooth-material interface showing elemental composition of the BioRoot RCS and the tooth. (Reproduced from Kebudi Benezra et al. [24] / with permission from Elsevier).
Since different methods of investigation were yielding contrasting results, the nature of the interaction of the hydraulic sealers with both coronal and root dentine was not clear. A further study using both confocal microscopy with a fluorescent dye added to the hydraulic cement and also using SEM showed that the addition of a fluorescent dye effected the penetration into dentine and also the physical and chemical characteristics of the materials [26, 27]. This was verified by SEM and bond strength testing of materials and materials doped with dye where the latter had higher bond strength and better interfacial characteristics than when the material was used without the dye as in a clinical situation [26]. Although interaction occurs between the materials and the tooth structure, the exact nature and particularly the role of silicon needs further investigation.
14.2.1.2 Hydraulic Cement Interaction with Blood and Tissue Fluids
The interaction of hydraulic cement with blood and tissue fluids has been likened to that of bioglass [28]. Bioglass interacts with synthetic tissue fluids and a layer of calcium phosphate is deposited on the material surface [29]. The interaction of hydraulic cement was shown to be similar as a layer of hydroxyapatite was shown to be deposited over the material surface in contact with synthetic tissue fluids [30–33]. The series of interactions have been called bioactivity and are shown in detail in Figure 14.4. The calcium and hydroxy ions released from the cement allow the interaction of phosphates in tissue fluids, and carbonated apatite is deposited over the material surface [29]. This deposition was also implicated in enhancing the push-out bond strength due to the interaction with the dentine [34].

Figure 14.4 A schematic of the five sequential stages of events that contribute to the manifestation of in vitro bioactivity of hydraulic calcium silicate cements after the latter is immersed in simulated body fluid (not to scale). ACP: amorphous calcium phosphate. (Reproduced from Niu et al. [28] / with permission from Elsevier).
Clinically, the free phosphate availability in blood and tissue fluids is limited. Characterisation of surface deposits over MTA derived from contact with subcutaneous tissues revealed the formation of calcium carbonate over the material surfaces [35]. The carbonate is derived from the carbon dioxide in the blood. Contact with blood clinically also caused the deposition of poorly crystallized calcium carbonate on the surface of hydraulic cement used as a root-end filling material [36]. The interaction with the blood when the cements were used for regenerative endodontic therapy also indicated the formation of carbonates [37]. The precipitate chemistry was also dependent on the type of synthetic tissue fluid used for testing [37]. It can thus be concluded that the bioactivity and precipitation of hydroxyapatite or amorphous calcium phosphate is an in vitro phenomenon as calcium carbonate preferentially forms in clinical situations.
The limitation of laboratory studies to provide any meaningful information related to clinical interaction of materials is highlighted in a review article [38]. The formation of phosphates on surfaces in clinical situations is also now debatable for bioglass where the role of carbonate formation is now also being recognised [39]. These surface deposits reduce the pH and this has clinical implications such as the annulment of the material antimicrobial properties [40], which can be detrimental when the hydraulic cement is used in bacteria-loaded areas such as root canals and caries-effected dentine.
Although contact with blood and tissue fluids has always been implicated with bioactivity, the wetting of the materials can also affect the physical properties. Blood contamination affects physical properties, dimensional stability, and colour. Similar effects have been reported with foetal bovine serum [41], and synthetic tissue fluids. Blood and tissue fluids inhibit the MTA hydration, and this results in the reduction or blocked formation of the calcium hydroxide, which is a by-product of cement hydration [42–44]. The retardation is induced by the formation of insoluble hydroxides in the alkaline solution that form a coating over the cement particles prohibiting further hydration. Hydraulic cement in contact with tissue fluids expands [45, 46]. The colour of the material changes in contact with blood with severe discolouration noted [35, 47].
14.2.2 Radiopacifier Characteristics
Radiopacifiers are added to hydraulic cements to enable radiographic detection postoperatively as the radiopacity of hydraulic cements is about 3 mm aluminium thickness [48, 49]. One of the preferred radiopacifiers for inclusion with hydraulic cements has been bismuth oxide. Bismuth oxide is present in 20% proportion in ProRoot MTA (Dentsply Sirona, Tulsa, OK, USA), which is the first hydraulic cement on the market. This 20% addition results in the MTA having a radiopacity of 6 mm aluminium thickness [7, 50, 51]. Although it was meant to be an inert additive, addition of bismuth oxide to the cement component caused changes in the physical properties [52–54]. The bismuth oxide leaches in increasing proportions [10] and it has been reported to move along the material to the tooth interface [55]. Due to its instability, it interacts with the surrounding tissues and fluids and this leads to material and tooth discoloration, which are discussed later in the chapter.
Due to the disadvantages of using bismuth oxide as a radiopacifier, a number of alternative compounds have been suggested [48, 56, 57]. These include calcium tungstate, zirconium oxide, tantalum oxide, and barium sulphate amongst others. A number of these compounds are used in commercially available products as indicated later. The 20% cement replacement with these alternative radiopacifiers does not produce comparable radiopacity to the same percentage replacement with bismuth oxide. Thus, the bismuth-oxide-free materials, although having a radiopacity value higher than 3 mm aluminium thickness suggested as a minimum by the International Standards Organization (ISO) for endodontic materials [58], still do not impart sufficient radiopacity to be detected well radiographically. Thus, the newer generation materials are considered to be inferior radiographically to the MTA containing bismuth oxide.
Due to the low level of radiopacity, most of the alternative radiopacifiers suggested in the literature require a larger amount of replacement of the cement component [48, 49, 56]. The different replacements of cement component can lead to changes in physical properties of the resultant material [52–54]. Replacement of the bismuth oxide with alternative radiopacifiers resulted in lower pHs, solubility, and an increase in setting [59]. The changes in the physical characteristics need to be interpreted with caution as the alternative radiopacifiers have different water demands, thus the effective water/cement ratio may be changed even though the water/powder ratio remains constant [49]. This is highlighted further by showing changes to material chemistry and physical characteristics when including additives and changing the radiopacifier [60].
14.2.3 Admixtures, Additives, and Vehicles
Besides the cement and radiopacifier, hydraulic cements in clinical use also include admixtures. These admixtures can be mineral or chemical. The mineral admixtures are usually in powder form and include a number of compounds that are added to the cement mixture to enhance its properties. One such compound is microsilica. Microsilica is used as a filler, but when added to hydraulic cements it also modifies the hydration reaction. In Equations 14.1 and 14.2, the hydration of tri- and dicalcium silicate results in the formation of calcium silicate hydrate and calcium hydroxide. If microsilica is present, the free microsilica will react with the calcium hydroxide to produce calcium silicate hydrate. These interactions have been reported extensively in the literature for hydraulic cement used in the construction industry [17–19] but also in the dental literature [61, 62]. This interaction is anticipated to enhance the physical characteristics by the further production of the calcium silicate hydrate; however, the elimination of the calcium hydroxide will have biological implications because the calcium hydroxide imparts the antimicrobial properties to the materials [63]. Clinical failure is mostly due to microbial recolonisation. Other additives are calcium oxide, calcium carbonate, and calcium phosphate. The reason for these additives is diverse. The calcium oxide is added to enhance the material activity at the early stages of the hydration reaction [50]. The calcium oxide is unstable, and in contact with water it will dissociate and release calcium ions in solution in an exothermic reaction, which will lead to kickstart of the hydration and a very high pH, which has been shown to affect the antimicrobial material activity [63].
The calcium carbonate is used as a filler and it serves the same purpose as the calcium oxide in releasing calcium ions in solution and aids the reaction kinetics [50]. The interactions of these additives are very specific and it is important not to ignore side reactions of other components interacting with the additives. Although the addition of calcium carbonate works well with tricalcium silicates, when the cement component is Portland cement, the formation of calcium hydroxide from the hydration reaction is compromised. With Portland cement, the formation of ettringite during hydration shown in Equation 14.3 is also affected by the presence of calcium carbonate. The sulphate ions are replaced by carbonate ions producing calcium carbosilicate and calcium carboaluminate [64]. Addition of calcium carbonate to MTA has also been shown to alter the physical properties, namely reducing the setting time, dimensional change, and compressive strength but increasing the solubility [65].
The calcium phosphate in various forms has also been used as an additive and because it is not a filler but an active compound, this renders the materials biphasic. The use of materials based on calcium phosphate results in the release of phosphate ions in solution which is meant to enhance the bioactivity of the materials and encourage the formation of amorphous calcium phosphate on the material surfaces. A 50–50% hydraulic cement and calcium phosphate monobasic or hydroxyapatite mixture was shown to lead to a depletion of the calcium hydroxide, which effected the biological properties [66]. This interaction was also dependent on the type of calcium phosphate used because the calcium phosphate monobasic led to a lower leaching rate when compared to the tricalcium silicate and the tricalcium silicate-hydroxyapatite mixture. The percentage addition also effected the interaction because a lower percentage of calcium phosphate monobasic did not interfere with the biological and antimicrobial properties [63].
A number of chemical admixtures are also used to enhance specific properties. These include calcium chloride, which is used to reduce the setting time but also reduces the solubility and increases the pH [67]. Other chemical admixtures are used to improve the material handling and in a number of water-soluble polymers [68, 69] they increase the flow and reduce the effective water to cement ratio, which results in enhanced physical characteristics but also may lead to cement retardation. The high plasticity cements also exhibit increased calcium ion release, which results in better biological characteristics [68]. Other chemical additives have specific scopes such as reducing washout, which is important in root-end surgery and perforation repair clinical applications. The MTA mixed with anti-washout gel exhibited lower levels of calcium ions in solution and reduced fluid uptake in the early stages of reaction. The anti-washout gel reduced the setting time of the cement and enhanced the compressive strength [70, 71 ].
For cement hydration, water is a necessary component as indicated in Equations 14.1–14.3. However, the setting time and the need to mix the components can be inconvenient in the clinical context. For this reason, the water has been replaced by alternative vehicles to enhance the clinical handling of the hydraulic cements. This has been done by developing premixed materials that use a non-water-based vehicle as this can be syringed directly to the site. These materials will then hydrate by imbibition of fluids from the environment [72]. These material types have been developed for root-end filling, for perforation repair, and as root canal sealers. The main shortcoming is that they may not set and this can be due to insufficient fluids available. Furthermore, hydraulic means the interaction with water; however, the fluid contact clinically is tissue fluids and blood. The phosphate present in blood may lead to retardation of material setting as indicated earlier [41–44].
Resin modified materials have been developed for pulp capping purposes and also as root canal sealers. Light curable hydrophilic resins replace the water used to mix the cement, thus the material is provided in a syringe and light cured allowing the material hardening. The uptake of fluids may not be enough from the dentine and pulpal fluids [73], and in fact this has been shown to compromise the chemical setting of the cement and the formation of the calcium hydroxide, which is needed for pulp healing [74]. A salicylate resin matrix has also been utilised for root canal sealers containing hydraulic cements. These systems have a paste/paste delivery. They require environmental fluids for setting and thus have the same limitations as the premixed systems [72, 75]. Furthermore, the release of calcium hydroxide from these sealer types has been shown to be much lower than that of the cement-water formulations and this compromises the antimicrobial activity [76].
14.3 Classification of Hydraulic Cements
Since the first hydraulic material has been developed and marketed, there have been a number of changes to the material chemistry and also a wider use of these material types. These materials can be classified according to their specific use and this classification are used later in this chapter to discuss the material properties. However, due to the several changes that have been undertaken to the material types; the known effects of these changes to the cement, radiopacifier, and vehicle; and the role of additives, a material classification based on the composition is proposed.
A classification of hydraulic cements based on their composition is shown in Table 14.1. Types 1–3 are Portland cement based. The Type 1 materials include Portland cement-based types that may or may not be radiopacified and which are mixed with water. MTA (ProRoot, Dentsply Sirona, Tulsa, OK, USA) and Medcem Portland (Medcem, Vienna, Austria) are Type 1 materials. The Type 2 materials are also based on radiopacified Portland cement using water as a vehicle but include additives that enhance the material performance. Brand names in this material type include MTA Angelus (Angelus, Londrina, Brazil), which includes calcium oxide added to enhance early age release of calcium hydroxide; Bio MTA+ (Cerkamed, Stalowa Wola, Poland), which has hydroxyapatite added; and MM-MTA (Coltène MicroMega, Besançon, France), which includes calcium carbonate as filler and calcium chloride accelerator. The role of the additives has already been discussed. While both Type 1 and 2 are water-based, Type 3 uses alternative vehicles. Commercially, such materials include TheraCal LC (Bisco, Schaumburg, IL, USA), which is a light curable resin-based material and MTA Fillapex (Angelus, Londrina, Brazil), a salicylate-based root canal sealer.
Table 14.1 Classification of hydraulic cements based on their composition.
Type | Cement | Radiopacifier | Additives | Water |
---|---|---|---|---|
1 | Portland cement | ✓/ X | X | ✓ |
2 | Portland cement | ✓ | ✓ | ✓ |
3 | Portland cement | ✓ | ✓ | X |
4 | Tricalcium/dicalcium silicate | ✓ | ✓ | ✓ |
5 | Tricalcium/dicalcium silicate | ✓ | ✓ | X |
The Types 4 and 5 reflect a change in the cement type. Rather than use Portland cement as a base, these material types are based on tricalcium and dicalcium cement. The main feature is the elimination of the tricalcium aluminate and the use of a laboratory-based source for the cement. The main difference between the Type 4 and 5 materials is the vehicle. Type 4 are water-based whereas Type 5 include alternative vehicles. The typical Type 4 materials are Biodentine (Septodont) and BioRoot (Septodont). EndoSequence (Brasseler, Savannah, GA, USA) and TotalFill (FKG Dentaire, La Chaux-de-Fonds, Switzerland) Biceramic Root Repair Materials are typical Type 5 materials.
The use of alternative cements to Portland cement has stemmed following concerns with the presence of aluminium and trace elements such as chromium, arsenic, and lead in the Portland cement [77–83]. Some companies are claiming heat treatment to industrial Portland cement, thus seeming to make it suitable for clinical use. The heat treatment does not reduce the amount of trace elements found in these materials, which have been claimed to be mobile and were traced in serum and main organs in animal models [84–87]. The presence of aluminium is counteracted by using tricalcium and dicalcium silicates and the trace elements by the manufacture of cements using laboratory-grade raw materials. MTA Angelus, although a Portland cement, has very low levels of trace elements [79] due to its in-house production by the company.
14.4 Specific Uses and Material Properties
Hydraulic calcium silicate cements can be used for various applications in endodontic therapies. This includes their coronal, intraradicular, and extraradicular use. For each application, specific material properties are discussed. These include the material chemistry, physical and mechanical properties, biological and antimicrobial characteristics, clinical use, and clinical interactions. The clinical success rates for each material types will also be discussed.
14.4.1 Application on the Coronal Pulp
The application of hydraulic calcium silicate cements on the coronal pulp includes their use for pulp preservation procedures, which encompasses indirect, direct pulp therapy and pulpotomy of permanent teeth (Figure 14.5). Furthermore, they are also used for apexogenesis procedures in immature permanent teeth (Figure 14.6) and as a barrier material in regenerative endodontic procedures (Figure 14.7).

Figure 14.5 Clinical case showing pulp preservation. Crown fracture with pulp involvement on the maxillary right central incisor of an 11-year-old girl (a–c). Periapical radiograph (a) and clinical images (b, c) taken at the first treatment session. The referring dentist had placed glass ionomer cement upon the pulp. There were no pain complaints and as the tooth reacted normally on carbon dioxide snow, vital pulp therapy was performed (d–g). After removal of the glass ionomer cement, the pulp exposure was visible (d). A Cvek preparation was performed until the cementoenamel junction (e), and the cavity was disinfected with sodium hypochlorite 3%. No excessive bleeding of the pulp was remarked, hence the cavity was cleaned with Tubulicid Blue Label (Dental therapeutics AB, Sweden) and Pure Portland Cement Med-PZ (Medcem GmbH, Switzerland) was placed upon the pulp (f). Subsequently, a glass ionomer lining was placed (g) and the tooth was immediately restored by means of a composite restoration (h–j). During the follow-up at (k) 12 and (l) 24 months post-treatment no clinical or radiographic signs were reported and the tooth reacted positively to carbon dioxide snow and electric pulp testing. Two years postoperative, aesthetically, the resin composite restoration was discoloured, but the tooth itself was not (m). (Courtesy of Nastaran Meschi, KU Leuven, Leuven, Belgium).

Figure 14.6 Clinical case showing an apexogenesis procedure. (a) Crown fracture with pulp involvement of the mandibular right lateral and central incisor of a 7-year-old boy. (b) The referring dentist had covered both teeth with glass ionomer cement, but it fell off several times. (c) There were no severe pain complaints (only cold sensibility on the uncovered incisor) and as the teeth reacted normally on carbon dioxide snow and had open apices, pulpotomy was performed in order to obtain apexogenesis. After removal of the glass ionomer cement, Cvek preparations were performed until the cemento-enamel junction and the cavity was disinfected with sodium hypochlorite 3%. No excessive pulpal bleeding was remarked, hence the cavities were cleaned with Tubulicid Blue Label (Dental therapeutics AB, Sweden) and the pulp was covered in both teeth with Biodentine™ (Septodont, France). The teeth were immediately covered with composite. Later on, both teeth were aesthetically restored by means of resin composite restorations (Courtesy of Dr. Pieter-Jan Swerts, KU Leuven, Leuven, Belgium). At the 1-year recall, (d) apexogenesis was obtained in both teeth. Clinically (e, f), no discolouration is visible. The mandibular right central insicor reacted positively to carbon dioxide snow, but the mandibular right lateral incisor did not (difficult to test under the resin composite build-up). Further follow-up is necessary. (Courtesy of Nastaran Meschi, KU Leuven, Leuven, Belgium).

Figure 14.7 Revitalisation procedure. (a, b) Crown fracture without pulp involvement and subluxation of both central incisors in the maxilla of an 8-year-old boy. The referring dentist restored both teeth with resin composite. However, the maxillary left central incisor lost its cold sensitivity and remained percussion sensitive. (c–e) As it was an immature tooth, a revitalisation procedure was attempted based on the Position Statement of the European Society of Endodontology [115]. (d) During the first (disinfection) session, vital pulp tissue was sensed in the apical third of the root canal. During the second revitalisation session, a blood clot was triggered periapically and upon this blood clot a sterile collagen scaffold (Collaplug, Zimmer Biomet, Germany) was placed. The root canal was sealed with Pure Portland Cement Med-PZ (Medcem GmbH, Switzerland). (e) The coronal part of the tooth was sealed with a glass ionomer cement lining and composite. The follow-up 3 (f), 6 (g), 12 (h, i), 24 (j, k), and 32 (l) months post-revitalisation revealed complete healing of the periapical lesion and a calcified root canal (h, j, and l). The tooth was slightly discoloured yellow (k, i) and clinically asymptomatic. However, it did also not react on carbon dioxide snow nor on the electric pulp tester. (Courtesy of Nastaran Meschi, KU Leuven, Leuven, Belgium).
14.4.1.1 Chemistry, Physical, and Mechanical Properties
Although MTA has been used for pulp capping and apexogenesis, it is not the ideal material for such clinical procedures. MTA is a Type 1 material, thus the setting time is not optimised, the strength is not adequate, and it is also very difficult to handle. Furthermore, MTA has been implicated with tooth discolouration, which is discussed later with the material interactions. The most important feature necessary for the coronal use of hydraulic calcium silicate cements is the calcium ion release, which although has been implicated with barrier formation and enhanced biological properties, seems to play a major role in antimicrobial characteristics [63].
The main breakthrough in the use of hydraulic cements for pulp preservation procedures is the controlling of the setting time. A lot of research has been undertaken to be able to reduce the setting time of water-based cements to clinically acceptable levels without compromising other material properties. These methods have included the use of additives such as calcium aluminate [61, 88, 89] and calcium chloride [67, 90–92] and also manipulating the cement manufacturing process and using a cement clinker rather than the actual cement itself [93, 94]. The clinker is the cement with the exclusion of the gypsum in the final process. Besides the cement acceleration, materials used for pulp capping and apexification procedures need to have adequate strength and microhardness. Improvement in such properties can be achieved by additives and admixtures. However, this can compromise the calcium ion release and thus the biological properties [67, 93, 95, 96].
The only hydraulic water-based material that has been specifically developed to be used for pulp preservation procedures is Biodentine. It is a Type 4 material, thus it is mixed with water for the hydration reaction. Biodentine has a specific chemistry; the powder is composed of tricalcium silicate, zirconium oxide, calcium carbonate, and some minor additives of iron oxide added to give the colour. The liquid is made up of water with some additions of calcium chloride and a water-soluble polymer. The powder is finer than other cement types of this category [50], and this ensures a higher reaction rate and the additives enhance the material properties.
Biodentine exhibits a higher initial rate of calcium ion release compared to other similar material types [97, 98]. This is due to the interaction of the calcium carbonate, which enhances the reaction rate [50]. The calcium chloride reduces the setting time considerably when compared to other similar material types [99]. The water-soluble polymer enables the reduction of the water to cement ratio, which in turn improves Biodentine’s physical properties. In fact, the compressive strength and micro-hardness of Biodentine are much higher than those reported for other similar material types [99]. The specific material chemistry, the fine particle sizes, the low water to cement ratio, and the presence of calcium carbonate all contribute to optimal materials properties aimed for clinical performance.
The setting of the material can be modified by the replacement of the water with a light curable polymer. The material will command cure and harden; the hydration reaction will depend on the imbibition of fluids from the environment through the material matrix. The same applies for the release of calcium hydroxide. One such material, which is clinically available, is TheraCal LC (Bisco, Schaumburg, IL, USA). Since when used for pulp-related procedures the amount of fluid is limited, there have been concerns on the performance of resin-based cements incorporating hydraulic cements. The ex vivo performance has been assessed using an entire tooth culture model where the dental pulp was kept vital by immersing the tooth in biologically active media [73]. The hydration of TheraCal LC was compromised in the ex vivo model indicating that the clinical material performance as a pulp capping material is limited [74].
14.4.1.2 Biological Characteristics
The biological characteristics are complex. Biodentine has been shown to cause specific pulpal reactions similar to those of mineral trioxide aggregate [100] with favourable cell proliferation and alkaline phosphatase activity of human dental pulp cells [101–103]. Biodentine allows reactionary and reparative dentinogenesis [104], which is essential for all pulp capping and apexogenesis procedures and has also been reported to enhance proliferation and odontoblast differentiation of human stem cells [104, 105] indicating its suitability as a barrier material in regenerative endodontic procedures. Biodentine allows the expression and release of dentine matrix proteins [106], has an anti-inflammatory potential, and induces the pulp regeneration capacity [107]. Furthermore, it enhances mineralised tissue formation [108–110].
Leachates of Biodentine are also biologically active [98]. The calcium releasing ability contributes also for the antimicrobial properties of Biodentine [63]. This property is important because dental caries is a bacterial induced disease. Biodentine is antimicrobial [111, 112]; however, the antimicrobial activity depends on the surface characteristics [113] and it is rendered ineffective against multispecies microcosm biofilm [114] indicating the need to reduce the microbial load prior to application of the material over the dentine. Although there is limited literature on cleaning of the caries effected dentine prior to material placement, the European Society of Endodontology (ESE) guidelines for the clinical management of the dental pulp [115] does recommend a wash with sodium hypochlorite (NaOCl) or chlorhexidine gluconate (CHX) to reduce the microbial load of the carious dentine.
The ESE guidelines [115] only recommend water-based hydraulic cements indicating that resin-based systems are inadequate for pulp management. Regardless of this, materials such as TheraCal are still used for pulp-related procedures. TheraCal has been shown to be cytotoxic [116] and its leachate also decreases pulp fibroblast proliferation and decreases expression of dentine sialoprotein and nestin. Furthermore, proinflammatory IL-8 release is released both in cultured fibroblasts and entire tooth cultures with significant tissue disorganisation [117]. The resin-containing materials shift the pulp response towards the inflammatory reaction while altering the regeneration process [107]. Another worrying feature about TheraCal in particular is the lack of information about the organic components present in the material [118].
14.4.1.3 Clinical Performance and Material Interactions
The hydraulic tricalcium silicate-based materials used coronally come in contact with various substrates when in clinical use. When used for pulp protection, they are in contact with dentine and dentinal fluids. These interactions have been discussed previously. The dentine is caries-effected dentine rather than sound dentine. Most of the research performed on bonding of material is performed on sound dentine [119–121] or using root dentine for testing materials bonded to coronal tooth structure, which is thus not clinically relevant. Adhesion to caries-effected dentine is challenging due to the microbial loading and the specific dentine microstructure. The use of NaOCl to condition the dentine prior to application of the hydraulic cements enhanced the bonding of the material to dentine [122
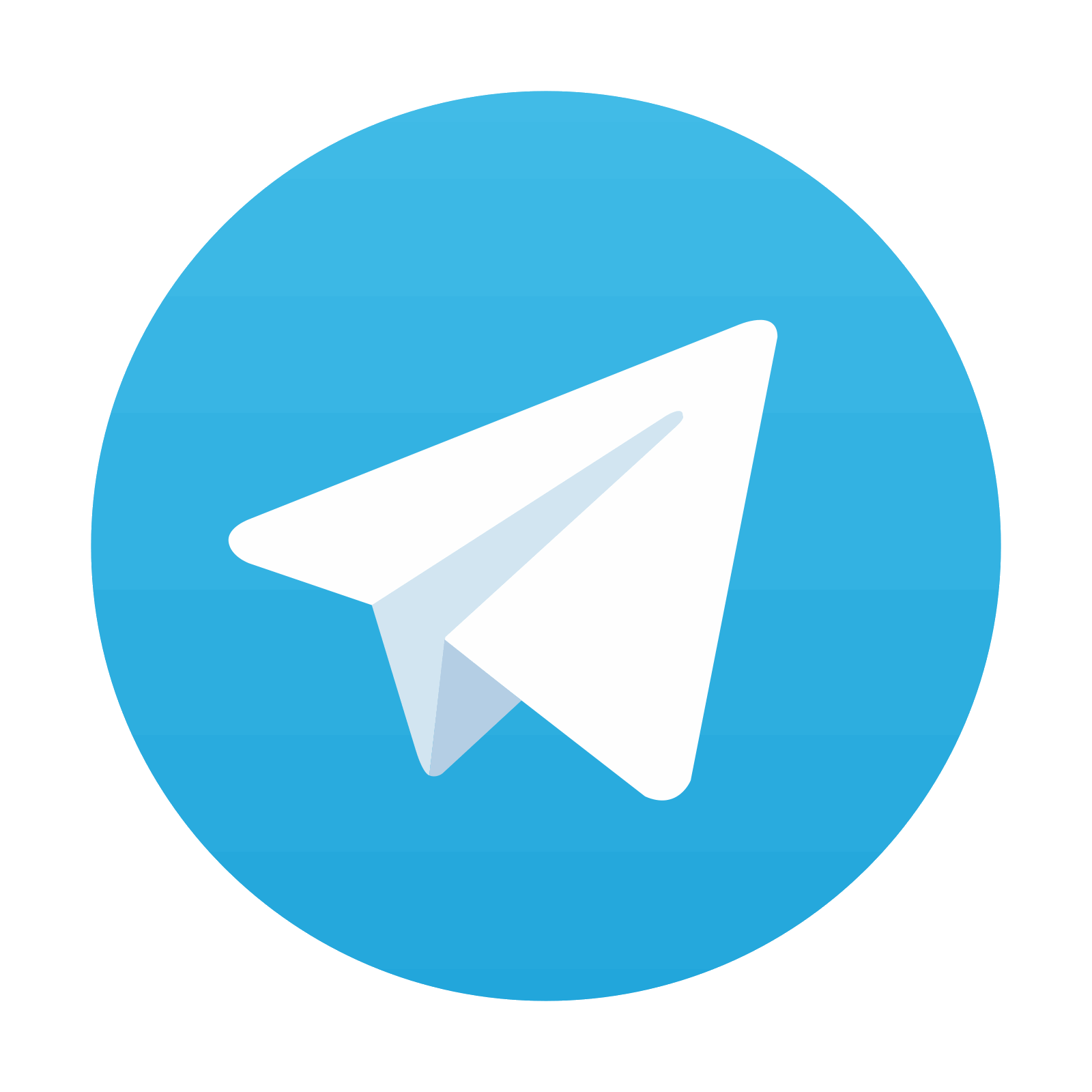
Stay updated, free dental videos. Join our Telegram channel

VIDEdental - Online dental courses
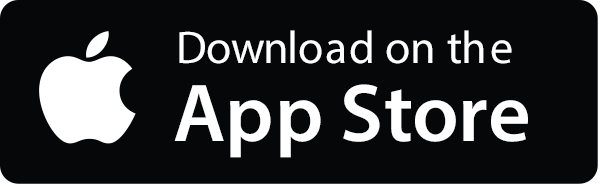
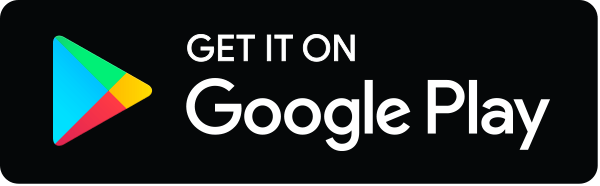