Head and neck and base of skull malignancies are challenging for surgical and radiotherapy treatment due to the density of sensitive tissues. Carbon ion radiotherapy (CIRT) is a form of heavy particle therapy that uses accelerated carbon ions to treat malignancies that may be radioresistant or in challenging anatomic locations. CIRT has an increased biological effectiveness (ie, increased cell killing) at the end of the range of the carbon beam (ie, within the target tissue) but not in the entrance dose. This increased biological effectiveness can overcome the effects of radioresistant tumors, tissue hypoxia, and the need for radiotherapy fractionation.
Key points
- •
Carbon ion radiotherapy is a form of heavy particle therapy with biophysical characteristics particularly suited for treating head and neck and skull base malignancies to provide improved disease control outcomes with reduced radiotherapy-associated side effects.
- •
Carbon ion radiotherapy has dosimetric advantages compared with x-ray radiotherapy that can reduce the dose to normal surrounding tissues.
- •
Carbon ion radiotherapy has increased biological effectiveness against the target malignancy, which can improve the effectiveness of treatment, particularly for radioresistant or hypoxic tumors.
- •
Clinical trials are underway to establish the benefits of carbon ion radiotherapy compared with x-ray and proton radiotherapy.
Introduction
Carbon ion radiotherapy (CIRT) has unique biophysical characteristics that are particularly advantageous for head and neck and base of skull (BOS) malignancies. The physical properties of carbon ions provide dosimetric advantages over conventional photon-based radiotherapy that can reduce the radiation dose distribution to nearby surrounding organs at risk. This is particularly relevant for malignancies in areas dense with radiosensitive normal tissues. Additionally, carbon ion radiation has increased radiobiological effects on the target tissues to improve the treatment response for radioresistant histologies. Herein, we describe the history of carbon ion therapy, the physical and biological characteristics of CIRT, and clinical data utilizing CIRT in head and neck and skull base malignancies.
A brief history of charged particles
Wilhelm Rontgen is credited with the discovery of x-rays in 1895 after he systematically analyzed and issued the first publication on the subject, describing x-rays as a new type of radiation. Rontgen identified the earliest medical use when he imaged his wife’s hand on a photographic plate using x-rays. The discovery of radioisotopes by Antoine-Henri Becquerel and Marie and Pierre Curie led to the earliest uses of x-rays and radioactive elements to treat cancer and by 1902, radiation was being used for cancer therapy for a variety of malignancies. During the next half-century, the use of radiotherapy for the treatment of malignancies expanded and so did the understanding of the potential benefits and risks of such treatments. By 1956, the first medical linear accelerator in the Western Hemisphere was installed at Stanford Hospital in San Francisco. With advances in harnessing the potential radioactivity and the development of accelerators to energize and target radiation, interest increased in the use of varying forms of radiation, particularly particle therapy. Robert Wilson at The Lawrence Berkeley National Laboratory (LBL) first suggested the potential clinical benefits of particle therapy (protons) based on their favorable dose distribution characterized by relatively low entrance dose at shallow depths and high energy deposition and rapid fall-off at the end of the beam range (see “Bragg peak” below). The difference in physical properties between charged particles and x-rays/photons forms the basis of the dosimetric benefits of particle therapies. In short, particles deposit most of their energy at the end of the beam path via a spike in energy deposition as the particle decelerates. This spike in energy deposition is termed the “Bragg peak,” after its discoverer, William Henry Bragg. Distal to the Bragg peak, there is little radiation dose deposition (exit dose). Upstream of the Bragg peak, there is relatively low-dose deposition. This is in contrast with electromagnetic radiation (x-rays and photons), which has no Bragg peak and deposit most of their dose outside of the intended target, including a significant dose upstream (entry dose) and downstream (exit dose) of the target. The LBL at the University of California, Berkeley, provided some of the earliest research and clinical experience with particle therapy. This included proton radiotherapy for intracranial targets beginning in the 1950s followed by helium ion therapy and additional charged particle therapy including neon, oxygen, nitrogen, and carbon from 1975 to 1992. The LBL charged particle program was terminated in 1992. In 1994, the Heavy Ion Medical Accelerator in Chiba opened in Chiba, Japan, and began treatment with carbon radiotherapy. This was followed by another heavy ion center in Hyogo, Japan, HIBMC (Hyogo Ion Beam Medical Center) and then GSI (Gesellschaft fur Schwerionenforschung) in Darmstadt, Germany, in 1997. At the writing of this article, there are 13 active carbon ion treatment centers worldwide, 9 in Asia (2 China, 7 Japan), and 4 in Europe (1 Austria, 2 Germany, 1 Italy). There are no carbon ion treatment facilities yet in North America. A carbon ion facility at Mayo Clinic in Florida, United States is currently under construction with plans to begin treatment in 2027.
The physical and radiobiological properties of carbon
A major difference in physical behavior that imparts an advantage of charged particle radiotherapy over electromagnetic radiation (ie, photons/x-rays) is the nature of dose deposition by charged particles, including the “Bragg peak.” All forms of radiation deposit energy along the beam path. Charged particles travel along their paths with energy deposition inversely related to the square of the velocity of the particle. Therefore, as the charged particle slows down in tissue, its energy deposition increases. Conversely, at shallow depths (ie, tissue surface) where the particle has its highest speed, there is relatively low energy deposition. As particles interact with tissue along their path, they slow down as they reach the end of their range and have a steep increase in energy deposition, resulting in an energy peak (ie, “Bragg peak”) followed by a sharp dose falloff immediately beyond the Bragg peak. The practical implication of this phenomenon is that there is little or no exit dose from charged particles. Fig. 1 shows radiation beam depth-dose profiles between photon and carbon ions and reveals differences between entrance doses and end of range doses between radiation sources. This difference in dose deposition between carbon and photons (x-rays) enables radiation delivery with reduced low and intermediate radiation dose to normal tissues surrounding the radiation target (via reduced entrance and exit dose). Although charged particles display generally similar dose distribution properties, there exist important differences between different particles, including the steepness of the Bragg peak and the steepness of the lateral dose falloff (ie, the amount lateral dose spread from the beam path). These differences between particles are due to differences in the particle mass and atomic number. The practical implication of this difference can be seen between 2 clinically useful particles—protons and carbon. Both are utilized in clinical practice and due to size and mass differences, they have different rates of distal and lateral dose fall-off (carbon has sharper dose falloff at both the distal and lateral beam edges).

Another significant difference between carbon and other types of radiation is the amount of energy deposited over the beam path. The term linear energy transfer (LET) quantifies the amount of radiation energy that is imparted over a distance in a medium (ie, tissue). Relative levels of LET are determined using gamma particles and x-rays as the reference. Carbon is a “high-LET” form of radiation and the LET of carbon is higher than that of protons, which is slightly higher than that of photons. High-LET radiation can have a higher impact on radioresistant tumors with increased cell killing. Additionally, high-LET radiation makes the biological impact of radiation less sensitive to cell cycle phase, tumor oxygenation, and radiation fractionation. Importantly, the LET of carbon is similar to photons along the flat portion of the dose deposition curve and only becomes high at the Bragg peak. This spares the normal tissues upstream of the intended radiation target from increased radiation treatment effect with high LET radiation and maximizes the impact of carbon radiotherapy on the target/tumor relative to normal tissues.
Another potential benefit of carbon ion radiotherapy is seen in preclinical studies suggesting increased immune response with combined CIRT and immune checkpoint blockade. , Additionally, there are data suggesting enhanced suppression of cancer migration and cancer cell invasion with CIRT compared with x-ray radiotherapy.
Clinical role for carbon ion radiotherapy in the head and neck and skull base
The most substantial expected benefits for CIRT include (1) the dosimetric advantages of sparing normal tissues from irradiation and (2) the increased biological effectiveness of carbon on the tumor. The dosimetric advantages of reduced normal tissue irradiation are particularly relevant in the head and neck/BOS areas where there is a density of radiosensitive normal tissues, including salivary glands, swallowing musculature, inner ear structures, optic apparatus, cranial nerves, brainstem, and cerebrum. These same structures render complete surgical resection of tumors in these areas difficult or impossible without significant morbidity. Therefore, radiotherapy is often necessary in the postoperative setting or even as part of definitive treatment. An additional challenge in these anatomic sites is the presence of histologies that are less radiosensitive, including adenoid cystic carcinomas (ACCs), chordoma, and chondrosarcoma. The increased biological effectiveness of CIRT is especially relevant for these histologies especially when they are incompletely resected, as is often the case. The next section describes clinical data for several such histologies in the head and neck and BOS for which CIRT shows promise.
Head and Neck/Skull Base Soft-Tissue Sarcomas
Soft-tissue sarcomas (STSs) are among the most attractive malignancies for treatment with CIRT due to their relative radioresistance and their requirement for aggressive oncologic resections. These considerations are particularly relevant for head and neck/skull base sarcomas where en bloc and negative margin resections are difficult or impossible and achieving local control with radiotherapy requires high doses. Due to the rarity of STS, there are limited data available with the use of CIRT. The facility in Chiba, Japan, prospectively observed 27 patients with unresected sarcomas of mixed histologies, including soft-tissue (16 patients) and bone sarcomas (11 patients), treated with 70.4 GyE in 16 fractions. With median follow-up of 37 months, 3-year local control was 91.8%. The local control rates for both STS and osteosarcoma in this study compared very favorably to historical controls at the same institution using lower doses of CIRT. Late radiation-related complications of Grade 3 or greater were observed in 23.1% of patients. Investigators at Gunma University Heavy Ion Center subsequently completed a prospective study including 10 patients with unresectable bone or STSs of the head and neck/BOS treated with 70.4 GyE in 16 fractions. They reported a 3-year local control rate of 72.9%.
Chordoma
Due to the high radiotherapy dose requirements to achieve local control and difficulty in achieving adequate surgical resection, skull base chordoma has long been a disease with indications for the treatment with particle therapy. , Skull base chordoma has similarly been a major focus of carbon ion radiotherapy facilities, and despite its rarity, there are several large retrospective series and a single-arm prospective observation study ( Table 1 ). Uhl and colleagues from the Heidelberg Ion Beam Therapy Center (HIT) retrospectively reviewed 155 patients with BOS chordoma treated with CIRT from 1998 to 2008. All patients had gross disease at the time of radiotherapy and received a median dose of 60 GyE in 20 fractions. With a median follow-up time of 72 months, they reported 5-year and 10-year local control rates of 72% and 54%, respectively. Mattke reported a follow-up series including 111 patients treated at HIT from 2009 to 2014 with BOS chordoma. Patients were treated to a median dose of 66 GyE in 22 fractions and with over 4years median follow-up had 5-year local control of 65%. Toxicity outcomes have not yet been reported from this series. The carbon ion facility in Pavia, Italy prospectively observed 135 patients with BOS chordoma treated with 70.4 GyE in 16 fractions. With a median follow-up of 49 months, 5-year local control was 71% and 3-year grade 3 or greater toxicity-free survival was 85%.
Institute | Design | Histology | Dates | Median F/U | No of Patients | Median Total Dose | Local Control | ≥Grade 3 Late Toxicity |
---|---|---|---|---|---|---|---|---|
HIT (Uhl, 2014) | Retro | Chordoma | 1998–2008 | 72 mo | 155 | 60 GyE/20fx | 5/10 y 72%/54% |
NR |
CNAO(Iannalfi et al, 2020) | Prospective | Chordoma | 2011–2018 | 49 mo | 65 | 70.4 GyE/16fx | 5y 71% | 3 y TFS 85% |
HIT (Mattke, 2022) | Retro | Chordoma | 2009–2014 | 52 mo | 111 | 66 GyE/22fx | 5y 65% | NR |
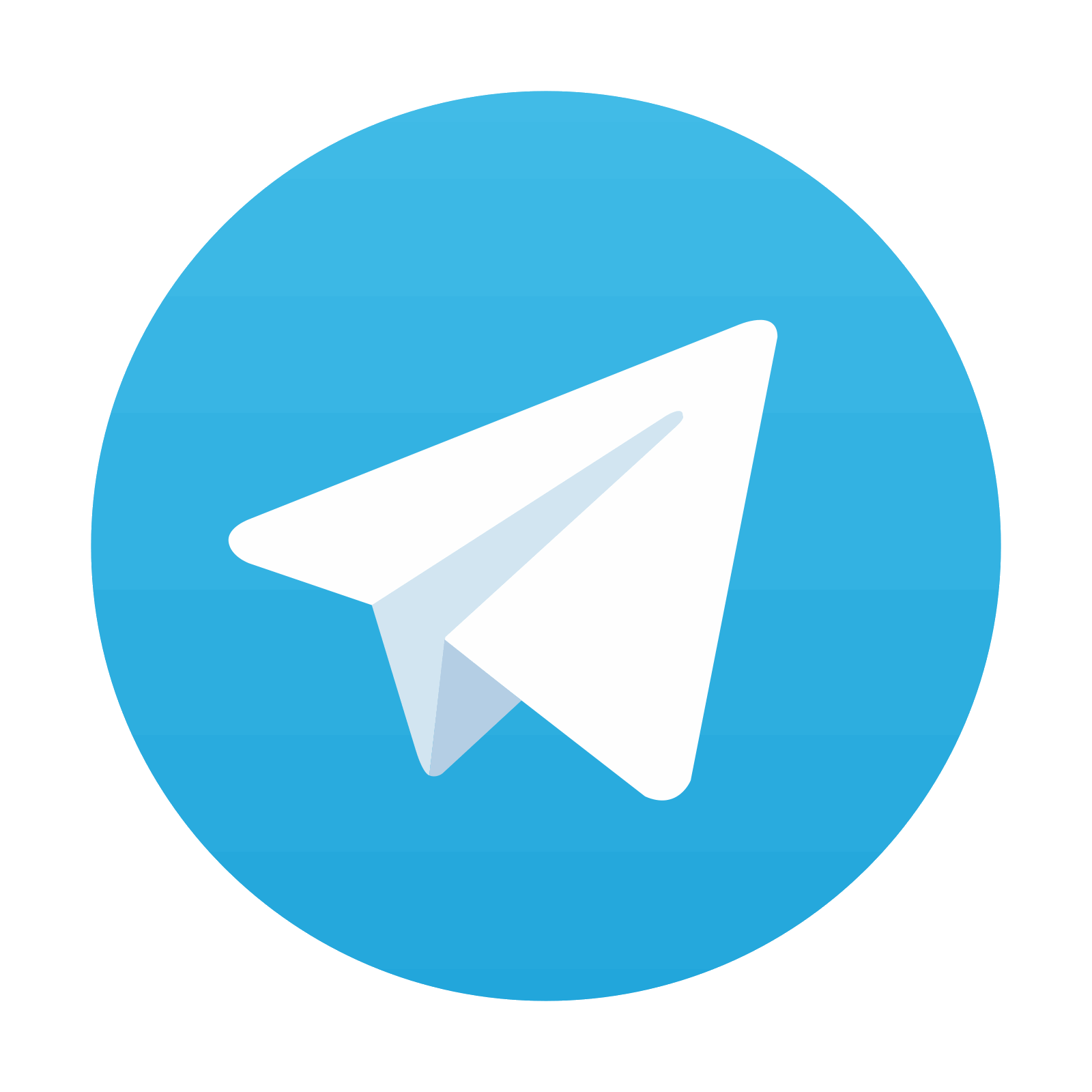
Stay updated, free dental videos. Join our Telegram channel

VIDEdental - Online dental courses
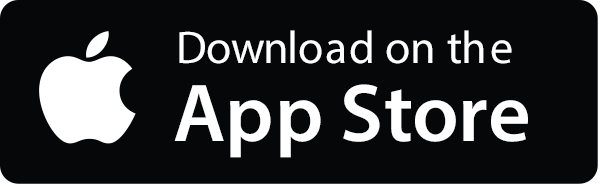
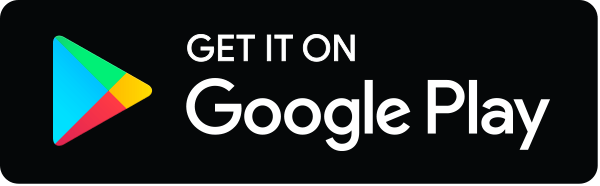
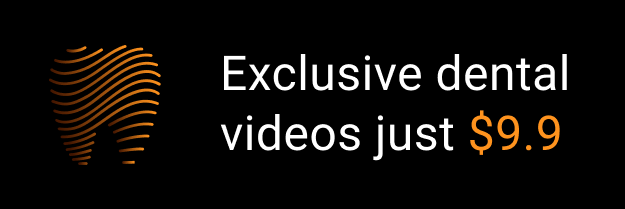