Abstract
Objectives
Despite developments in polycrystalline ceramics, glassy dental-ceramic materials provide the optimum cosmetic option in most clinical situations to mimic the natural dentition. The clinical success of glassy dental-ceramic materials is often attributed to resin-adhesive bonding techniques. In this study we explore whether shrinkage stresses generated on photo-polymerisation of the resin-cement are sufficient to induce ceramic surface defect stabilization, and we quantify the transient nature of the induced stresses.
Methods
Stress-induced changes in a feldspathic ceramic over a range of thicknesses (0.5–2.0 mm: n = 20 per thickness) were measured using a profilometric technique at baseline for each disc-shaped specimen (mean of the maximum deflection ( δ baseline )) and again following polymerisation of a controlled resin-cement thickness on the contra-lateral surface. Measurements were repeated at 30, 60, 90 and 1440 min following photo-polymerization ( δ 30 , δ 60 , δ 90 and δ 1440 , respectively) before bi-axial flexure strength (BFS) determination at 24 h.
Results
A repeated measures ANOVA and post-hoc Bonferroni tests determined that δ 1440 was significantly different from δ baseline (p = 0.02), δ 30 (p < 0.01) and δ 60 (p < 0.01) but not δ 90 (p = 0.61). Data exploration revealed that there were differences in directionality of the independent variable (mean of the maximum deflection ( δ )) with a proportion of specimens increasing in deflection and others reducing. The directionality of the effect strongly correlated with the BFS values. Where δ 1440 – δ baseline was low and/or negative, BFS values were also low. High BFS values were observed when δ 1440 – δ baseline was large and positive (indirectly inferring high shrinkage-stress-induced-deformation).
Significance
A link between shrinkage stresses associated with the photo-polymerization of dental resin-based cements and the reinforcement of dental porcelain has clearly been established.
1
Introduction
In 2002, a review entitled ‘Are adhesive technologies needed to support ceramics? An assessment of the current evidence’ concluded that resin-cements reinforce dental ceramics, however, the assessment of the ‘available evidence’ was poor . Of the 28 studies reviewed , only two were direct clinical comparisons with the remainder based on supposition, inconclusive in vitro mechanical tests including load-to-failure studies of adhesively cemented, anatomically representative restorations. Unfortunately, the dental literature is replete with ‘crunch-the-crown’ load-to-failure studies despite fracture behavior in such tests being dominated by the indenter-contact surface which fails to simulate clinical failure modes . Load-to-failure studies of anatomically representative restorations add little to the mechanistic understanding of resin-cement strengthening and cannot be used to guide materials-development or inform the profession . From the results of the two direct clinical comparison studies , it has become accepted that adhesive cementation of monolithic dental-ceramic restorations fabricated from glassy/glass-ceramic substrates is essential to optimise restoration longevity. When adhesively coupled to the underlying tooth substrate, relatively ‘weak’ ceramic materials demonstrate significantly higher clinical survival rates compared with acid-base cement systems . This consensus was predicted by John McLean who postulated that the tooth could act as a ‘reinforcing core’ for ceramic restorations with the adhesive resin-cement providing a ‘synergistic bond’ to confer system strength . Despite the mechanical advantages of polycrystalline ceramics , glassy ceramics still provide the optimum minimally invasive cosmetic option for the clinician to mimic tooth structure in terms of form, shade, translucency, lustre and hue . It is for this reason that most polycrystalline ceramics have to ‘veneer’ the core material with porcelain which has subsequently become the mechanical ‘weak-link’ . Extrapolating from McLean’s postulation, two questions arise: (1) are we close to optimising the ‘synergism’ between the ceramic/resin-cement/tooth substrate complex? and (2) with appropriate technological development could reinforcing ceramic cores be eliminated completely from the prosthodontists armamentarium to herald a truly conservative all-ceramic indirect restoration?
To date, the way in which resin-cements reinforce ceramics remains poorly understood and studying the discrete components or model analogues of the ceramic/resin-cement/tooth substrate complex, in isolation and/or in combination, has been shown to be a useful in vitro approach . When considering the fracture resistance of glass bonded to a relatively compliant polymer substrate, Lawn et al. identified competing fracture modes, with fracture originating either from contact zone cone cracks during testing or from radial fracture at the glass-substrate interface. The observation of the former is encouraging as it demonstrates that it is possible to reinforce the glass sufficiently against radial failure so that contact damage failure predominates. Further studies have focused on the resin-cement/ceramic interface and identified the magnitude of resin-strengthening is sensitive to the pre-existing ceramic surface defect population . Different magnitudes of strengthening have been observed to be dependent on: the size of the critical ceramic surface defect ; the nature of bonding at the resin-cement/ceramic interface including silane coupling agents with pre-test storage (hydration and time) regime ; and the modulus of elasticity of the resin-cement .
One concept that has been minimally explored is whether resin-cementation of dental ceramic restorations confers compressive stabilisation of the ‘fit’ ceramic surface. In 1993, Nathanson suggested that the stresses generated due to the volumetric shrinkage associated with photo-polymerisation of methacrylate resin-based cements could exert a stabilising compressive stress over the surface flaw integral, thereby resulting in an increased energetic requirement to reach the critical tensile stress in the ceramic to initiate catastrophic failure . The purpose of the current study was to first ascertain whether the shrinkage stresses generated on initial resin-cement photo-polymerisation are sufficient to confer surface defect stabilisation and secondly to understand the transient nature (or not) of the residual stresses conferred post photo-polymerisation of the resin-cement.
2
Materials and methods
2.1
Specimen manufacture
VITA VM7 (Vita Zahnfabrik, Bad Säckingen, Germany) disc-shaped specimens (13.8 mm nominal diameter) were fabricated by manipulating a porcelain slurry prepared to a powder/liquid mixing ratio of 0.3/0.11 g/mL of VM7 base dentin powder (Lot 7433)/modelling fluid (Lot 4209R) into Nylon ring-moulds (0.7, 1.2, 1.7 and 2.2 mm thicknesses: n = 20 per thickness) secured to a burnished aluminum baseplate . The mould assembly was vibrated (Croform Techniques Ltd, London, UK) for 90 s and excess liquid was repeatedly removed using absorbent tissues . Following condensation, surplus material was removed with a razor blade, and discs were sintered on a silicon-nitride firing-slab in a vacuum-furnace (Vita Vacumat 40, Vita Zahnfabrik, Bad Säckingen, Germany) which involved pre-heating to 500 °C for 420 s, before the temperature was increased at 55 °C/min to 910 °C under vacuum, held for 60 s and allowed to slow cool to room temperature (Vita VM7 Product Specification, 2016).
Both sides of the disc-shaped specimens were wet-polished for 90 s with increasing grades (from P320 to P600 and P800) of silicon carbide abrasive papers (Buehler, Lake Bluff, IL, USA) on an Alpha and Beta Grinder-Polisher (Buehler) at 100 rpm under a specimen force of 3.3 N, with an exchange of abrasive paper at 30 s time-intervals . Specimen thicknesses were periodically measured with a digital micrometer (Digimatic Micrometer, Mitutoyo Corp., Tokyo, Japan) to create a distribution of disc-thicknesses ranging from 0.5 to 2.0 mm. The sintering of specimens at different material thicknesses (and therefore volumes) and the subsequent surface polishing introduces differences in residual stress state between individual disc-shaped specimens. To account for this, discs were annealed by heating from 200 to 610 °C in air at 20 °C/min, held at 610 °C for 40 min before cooling to 60 °C at 2.9 °C/min . To create a surface conducive to profilometric evaluation of stress-induced-distortion in the disc-shaped specimens, one surface of each disc was further wet-polished with P1200, P2400 and P4000 abrasive papers under the conditions outlined above (100 rpm, at 3.3 N specimen force for 90 s time-intervals with abrasive paper exchange every 30 s). The contralateral surface of each disc-specimen was then air abraded with 50 μm diameter alumina particles from a distance of 1 cm, at a 90° angle of incidence and 2.5 bar pressure to produce a surface roughness conducive to bonding while imparting a consistent surface defect population . The final disc-thicknesses were re-determined with the digital micrometer. Additionally, a further 20 disc-shaped specimens were fabricated using the 1.2 mm mould and finished to a 1 mm thickness using polishing regime outlined above while maintaining the identical annealing and air abrasion regimens employed to act as an un-cemented (control) group for bi-axial flexure strength (BFS) determination.
2.2
Deflection determination
The disc-shaped specimens were aligned on a three-axis levelling-device where a contact diamond stylus profilometer (Talysurf CLI 2000, Taylor-Hobson Precision, Leicester, UK) with a 90° conisphere stylus tip (2 μm radius) was used to perform 126 measurement traces across a 5 mm 2 area (10 mm length and 0.5 mm width) coincident with the center of the polished specimen surface. The area of interest (10 mm length and 0.5 mm width) was marked to ensure the consistency of subsequent repeat measurements . Measurements were performed at a stylus velocity of 1 mm/s and an applied force of 0.75 mN, with a 4 μm step-size (y-direction), recording data points every 10 μm (x-direction) at a 40 nm resolution (z-direction). The mean of the maximum deflection ( δ ) was determined as the mean of the maximum central z-vector relative to the disc-periphery (μm) to provide an initial baseline (pre photo-polymerisation) measurement ( δ baseline ).
The alumina-particle-air-abraded surface of each disc-shaped specimen was primed with 3-methacryloxypropyltrimethoxysilane (Rely-X Ceramic Primer, 3 M ESPE) and allowed to air-dry (23 ± 1 °C at 50 ± 1% relative humidity) for 10 min. To standardise the cementation process and generate a consistent resin-cement thickness, 0.035 g of Rely-X Veneer Cement (3 M ESPE) was deposited onto the center of the primed-disc , covered with a thin acetate sheet and a 1 mm thick glass slide and pressed under a standard load until the resin-cement spread to the disc-periphery. The resin-cement was light-irradiated using an Optilux 501 light-curing-unit (SDS Kerr, Danbury, CT, USA) for 20 s at a light intensity of 740 ± 38 mW cm −2 with a 13 mm light-tip-diameter, held against the glass slide during photo-polymerisation. The thicknesses of the resin-cemented discs were determined at three separate points and the mean resin-cement thicknesses calculated. Repeat profilometric measurements were performed over the measurement track at 30, 60, 90 and 1440 min following photo-polymerisation (using the protocol employed above) to obtain the mean of the maximum deflection values (μm) namely δ 30 , δ 60 , δ 90 , and δ 1440 , respectively. All measurements were performed at room temperature of 23 ± 2 °C and a relative humidity of 50 ± 10%.
Immediately following the determination of δ 1440 , the BFS ( σ ) of all disc-shaped specimens were determined using a ball-on-ring assembly where the resin-coated-discs were positioned centrally on a knife-edged-support (10 mm diameter) with the polished surface uppermost and loaded with a stainless-steel spherical ball-indenter (4 mm diameter) at 1 mm/min. σ was calculated at the disc-center at axial positions throughout the specimen thickness ( z ), with the bonded interface at z = 0 , the ceramic surface at z = t 1 and the resin-surface at z = −t 2 using the approach described by Hsueh et al. .
σ = − 3 P ( 1 + ν ) ( z − t n ) 2 π ( t 1 + t 2 ) 3 [ 1 + 2 ln ( a b ) + 1 − ν 1 + ν ( 1 − b 2 2 a 2 ) a 2 R 2 ] [ E 1 * ( E 1 * t 1 + E 2 * t 2 ) ( t 1 + t 2 ) 3 ( E 1 * t 1 2 ) 2 + ( E 2 * t 2 2 ) 2 + 2 E 1 * E 2 * t 1 t 2 ( 2 t 1 2 + 2 t 2 2 + 3 t 1 t 2 ) ]
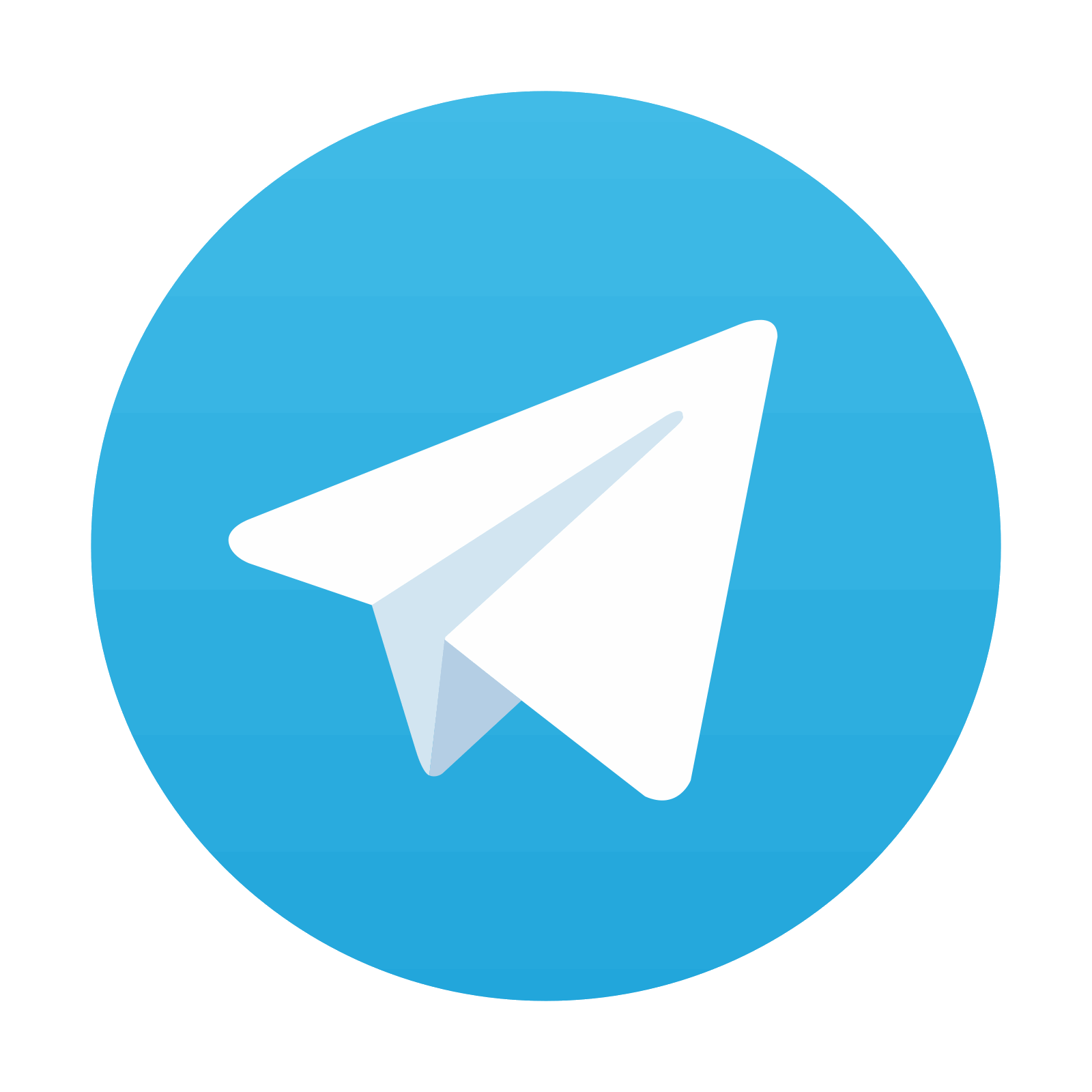
Stay updated, free dental videos. Join our Telegram channel

VIDEdental - Online dental courses
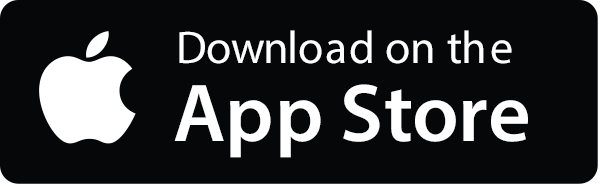
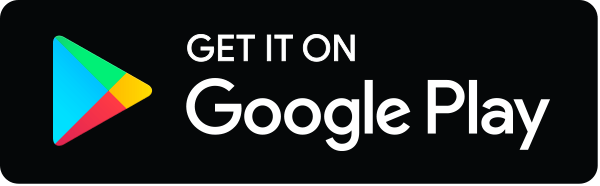