Abstract
Objective
The objective of this article is to describe the importance of fatigue to the success of restorative dentistry, with emphasis on the methods for evaluating the fatigue properties of materials in this field, and the durability of their bonded interfaces.
Methods
The stress-life fatigue and fatigue crack growth approaches for evaluating the fatigue resistance of dental biomaterials are introduced. Emphasis is placed on in vitro studies of the hard tissue foundation, restorative materials and their bonded interfaces. The concept of durability is then discussed, including the effects of conventional “mechanical” fatigue combined with pervasive threats of the oral environment, including variations in pH and the activation of endogenous dentin proteases.
Results
There is growing evidence that fatigue is a principal contributor to the failure of restorations and that measures of static strength, used in qualifying new materials and practices, are not reflective of the fatigue performance. Results of selected studies show that the fundamental steps involved in the placement of restorations, including the cutting of preparations and etching, cause a significant reduction to the fatigue strength of the hard tissue foundation. In regards to the bonded interface, results of studies focused on fatigue resistance highlight the importance of the hybridization of resin tags, and that a reduction in integrity of the dentin collagen is detrimental to the durability of dentin bonds.
Significance
Fatigue should be a central concern in the development of new dental materials and in assessing the success of restorative practices. A greater recognition of contributions from fatigue to restoration failures, and the development of approaches with closer connection to in vivo conditions, will be essential for extending the definition of lifelong oral health.
1
Introduction
The failure of structural materials that are designed to withstand mechanical loading can result from a variety of causes. While failures associated with overloads are generally considered first, fatigue is more often the primary mode of failure of load-bearing structures . Fatigue is regarded as the reduction in load-carrying capacity of a material subjected to cyclic stresses and results from an accumulation and growth of damage. As such, conditions that involve repetitive loads warrant consideration of fatigue-related material failures. The cyclic nature of mastication, and the delayed failure of restorations after a period of oral function, clearly suggest that an understanding of fatigue is relevant to the success of restorative dentistry.
A highly cited study concerning the annual incidence of fracture in the United States estimated a cost of 119 billion dollars (in 1982 value), which equated then to about 4% of the gross national product . The investigation emphasized that this cost could be significantly reduced by including more emphasis on design for resistance to fatigue failures. That could be an equally relevant message for the field of dental materials. But what relevance does a study focused on engineering structures have to the field of dentistry?
The two major reasons for restored tooth failure are recurrent caries and fractures . The replacement of failed restorations consumes the majority of a dentist’s daily activities. In fact, replacing failed restorations accounts for 50–70% of all clinical work performed . This is an important point, as the cost for tooth cavity restorations in the U.S. was nearly $50 billion in 2005 alone . If even only 10% of restoration failures were attributed to fatigue and could be avoided by better understanding, that would accumulate to an annual savings of billions of dollars. The repair of failed restorations frequently involves the removal of tooth structure and a reduction of the hard tissue foundation available for supporting the subsequent restoration. That reduces the chance of success.
It is essential to acknowledge that fatigue is not a new topic in dentistry. Studies in this area began to appear in the late sixties . In fact, the fatigue properties of dental composites have received considerable attention for over two decades . In vitro studies seldom take into account the contributions of fatigue and aging factors, despite their contributions to the formation of cracks and their propagation within the materials placed in the mouth . Yet, this topic appears to be gaining recognition. Indeed, fatigue is now recognized as either the primary mode of failure or a contributing mechanism in the failure of both direct and indirect restoratives .
Fatigue studies involve the application of cyclic loading and require considerably more time than standard strength tests. There is also a general belief that the fatigue strength of a material can be obtained from the static strength, which could reduce the incentive to perform evaluations involving fatigue. This relationship is limited primarily to metals and not necessarily true for other material classes. Indeed, the conventional bulk properties of dental resin composites including the elastic modulus, flexural strength and fracture toughness are reportedly not good indicators of the fatigue resistance and could have limited clinical relevance. Furthermore, recent assessments of in vitro evaluations and clinical outcomes suggest that the fatigue properties of resin composites may be useful in predicting clinical performance . These emerging findings suggest that the fatigue properties of dental materials, and the durability of bonds that maintain them in place, should be considered important metrics of performance.
It is not always clear how fatigue studies should be performed, and what can kind of understanding can be garnered from these evaluations. The objective of this article is to convey the significance of fatigue to the success of restorative dentistry through some examples, with emphasis on the methods for evaluating the fatigue properties of materials and their bonded interfaces, as well as the concept of durability.
2
Background
There are many aspects of fatigue failures that are unique from those caused by static loading. The first and most important characteristic of fatigue is that failures occur at stresses that are generally much lower than the static “strength” as defined by a yield or ultimate strength. As such, cyclic loading facilitates failures that are not adequately predicted by measures of static strength. Secondly, fatigue is a stochastic process that is dependent on the growth and coalescence of intrinsic flaws in the material. That indicates that the fatigue strength is not deterministic because the mechanical response is largely dependent on conditions or processes that influence the internal flaw distribution and flaw sizes. Through the energetics of the cyclic loading process, the flaws grow to a size that reduces the ability to bear load. The third quality is that fatigue of a structure is more sensitive to changes in the surface quality than other aspects of mechanical behavior. And the fourth aspect of importance is that the fatigue resistance of a material is more likely to be influenced by other contributing forms of degradation (synergistic effects). For example, corrosion of metals is a well-known surface phenomenon that can reduce the fatigue strength and together they operate to accelerate the process of degradation .
These special qualities of fatigue are highly relevant to the success of restorations. Cutting of the preparation and the additional steps including etching, application of a primer, etc. contribute to both the surface quality and surface integrity of the materials serving as the foundation for adhesive bonds. In addition, the structure of these bonds is complex. Bonding to dentin and enamel involves at least three distinct materials, including the restoration, the resin adhesive and the hard tissue, as well as the interfaces between them. The difficulties realized in the placement and potential curing of the restorative materials increases the likelihood of introducing defects and their average size. Depending on the preparation and the mechanisms enrolled in anchoring the restoration, there are hybrid layers and interdiffusion zones with their own unique microstructure and properties. Consequently, the fatigue strength of the adhesive bond is controlled by all of these contributing elements. Identifying the weakest link is critical to the design and development of improved materials and practices that will extend the longevity of restorations.
Considering that the magnitude of cyclic stress borne by the forces of mastication are small enough to avoid bulk fracture, then there are two potential modes of fatigue failure. The first mode is where failure originates from existing inherent defects in the material ( Fig. 1 a). These defects coalesce with cyclic loading and facilitate fracture through a reduction in capacity to bear load. This process is regarded as “stress-life fatigue” and is the most common mode of fatigue. Alternatively, fatigue failures can originate from a well-defined flaw or crack ( Fig. 1 b) that undergoes extension via cyclic loading until it reaches a length that permits fracture due to the magnitude of the stress intensity. This process is regarded as fatigue crack growth. Both of these modes of fatigue are relevant to dental materials and the practice of restorative dentistry. Contact fatigue and dynamic fatigue (or slow crack growth) are also important, primarily relevant to crown materials, and warrant separate treatments.
2.1
Stress-life fatigue
The stress-life fatigue behavior of a material is evaluated by subjecting specimens with well-defined geometry to cyclic loads that result in a desired magnitude of cyclic stress. While standards are widely available that guide the choice of specimen geometry and size for most engineered materials, there are limited testing standards for dental materials. Cyclic loading of the specimens is conducted via tension or flexure at an acceptable frequency, and the number of cycles to failure is documented. Flexure is often preferred as it generates a simple stress state and reduces the problems associated with gripping and alignment of specimens that is associated with tensile loading.
If the goal of fatigue testing is to understand the average stress that results in a specific finite “life”, e.g. 50k cycles to failure, then the applied load is adjusted in subsequent tests according to the staircase method to distinguish the corresponding stress amplitude that results in the life of question. However, if the goal is to understand the fatigue strength distribution over a range in stress that results from oral function, then a fatigue life diagram is developed. Fatigue life diagrams describing the fatigue strength distribution of human coronal dentin for two donor age groups are shown in Fig. 2 a. One benefit of constructing the fatigue life diagram is that the data can be modeled with a simple power law model ( Fig. 2 a), which describes the fatigue strength distribution over the entire range of finite life. The diagram also helps distinguish if the material exhibits a fatigue limit (S e , also termed endurance limit), which is the cyclic stress amplitude below which the material exhibits a tremendously long life (does not fail).
Considering that the average number of masticatory cycles ranges between one and two thousand per day , the fatigue life diagram and determination of the endurance limit should be customary for dental materials, despite requiring a greater investment of time. For example, in comparing the fatigue strength distributions of young and old dentin in Fig. 2 a, a comparison at a life of 1k cycles shows that the fatigue strength of the old dentin is roughly 25% less than that of young dentin. That comparison can be performed for any finite life, and as evident from the distribution in Fig. 2 a, the relative fatigue strength of old dentin decreases with longer definition of life. A comparison of the fatigue limits (S e ) indicates that the fatigue strength of old dentin is nearly 50% lower than that of young dentin . If the goal of restorative dentistry is to promote life-long oral health, then the comparisons using the endurance limit are most relevant. They are also most objective. Finite life comparisons are valuable as well, but they provide an incomplete picture, and sometimes even misleading view, of the fatigue response.
2.2
Fatigue crack growth
The fatigue crack growth resistance of a material is determined by subjecting specimens with well-defined geometry and a sharp crack to cyclic loads that results in incremental crack extension. There are no testing standards explicitly for dental materials that guide the choice of specimen geometry and size to use for fatigue crack growth evaluations. A number of different specimens have been used for this purpose . Fatigue crack growth evaluations on dental materials are often conducted using beams loaded in flexure or Compact Tension (CT) specimens , to generate cyclic tensile stresses that achieve an adequate stress intensity range for cyclic extension of the crack. Cyclic loading of the specimens is conducted at an acceptable frequency and the crack length is measured after specific intervals of loading. The history of crack extension is then evaluated using a fatigue crack growth diagram, where the average growth rate (Δa/ΔN) is plotted in terms of the average stress intensity range (ΔK) over the increments of cyclic extension.
Similar to the comparison of fatigue strength distributions in Fig. 2 a, a fatigue crack growth diagram for young and old dentin human coronal dentin is shown in Fig. 2 b. This data was obtained for cyclic crack growth perpendicular to the dentin tubules ; the definition of the two age groups is consistent with that used for the stress-life responses ( Fig. 2 a). The cyclic crack growth history within a single specimen of young coronal dentin (Young*) is highlighted to enable observation of how cyclic crack extension progresses. Although the growth response of each specimen can be quantified in terms of the classical initiation and steady-state growth behavior , they are not really necessary to show the utility of this approach. Details of quantitative treatments applied to biomaterials are available elsewhere .
The fatigue crack growth diagram in Fig. 2 b presents the responses for specimens obtained from the teeth of many young and old donors. The distribution of this experimental data shows that even within the young and old age groups that there is considerable variation in the responses. This variation is largely related to the spatial variations in microstructure of coronal dentin . Fatigue crack growth diagrams are useful as they enable simple comparisons of the resistance to cyclic crack growth. Responses to the lower right in this diagram signify a material with higher resistance to fatigue crack growth, while those to the upper left undergo fatigue crack growth more easily, as highlighted with “+” and “−” symbols in Fig. 2 b. It is clear from this general description that old dentin exhibits substantially lower fatigue crack growth resistance than that of young dentin. It is also evident that the initiation of fatigue crack growth from existing cracks starts at much lower stress intensity range in old dentin. A comparison of the average growth rates can be made as a function of the stress intensity range. Choosing a value of ΔK that is within the range of that generated by cyclic mastication , it is possible to compare the average steady-state cyclic crack growth rates, which is shown in Fig. 2 b for ΔK ≈ 0.75 MPa*m 0.5 . The ratio of the cyclic growth rates at this level of stress intensity for old and young dentin exceeds 100, indicating that cracks in old dentin grow at 100 times the rate of those in young tissue. According to the reciprocal of this value, the corresponding “life” of old teeth with cracks subjected to the same level of mastication would be less than 1% of the life of a young tooth. This simple comparison shows that substantial degradation that can occur from the introduction of cracks within teeth during restorative processes, and that this damage is much more detrimental with increasing patient age.
2.3
Some relevant examples
With this basic understanding of how to test and characterize the fatigue and fatigue crack growth behavior of materials, it is possible to address some factors relevant to dental practice. The first concern is the importance of introducing the cavity preparation and surface quality. As previously highlighted, fatigue responses are sensitive to the surface quality of materials. Finishing of the margins and polishing of the occlusal surface are considered important steps in the placement of direct restorations. But are these steps really important to the strength and longevity of the restoration?
Lohbauer et al. reported that surface texture is important to the strength of resin composites and glass ceramics, even under monotonic loading. For surfaces with average roughness (Ra) larger than 2.1 μm, flaws created by the surface treatments were large enough to have a significant effect on the strength. In regards to cutting the cavity, Staninec et al. showed that laser preparations introduced cracks in dentin that exceeded 100 μm in length under some treatment conditions and caused a significant reduction in strength. But the static strength of a material is generally less sensitive to surface condition than the fatigue strength. Indeed, Majd et al. reported that while there was no influence of burs or airjet surface treatments on the strength of dentin under quasi-static loading, both preparations caused significant reductions to the fatigue strength.
Cutting the preparation and etching are integral steps in the placement of resin composite restorations. Lee et al. evaluated the effects of cutting and etching on both the static and fatigue strengths of coronal dentin. The average flexure strength of coronal dentin specimens prepared using diamond abrasive slicing equipment (control) was 154 ± 24 MPa. It was found that there was no difference (p > 0.05) in the strength between these “flaw-free” controls and specimens prepared using bur treatment or etching treatments. Hence, there were no effects of bur cutting or etching to the static strength of dentin.
A comparison of the fatigue life distribution for the control specimens (prepared by diamond abrasive slicing) with specimens that were prepared using the bur treatment (BT) is shown in Fig. 3 a. Similar to the fatigue life diagram in Fig. 2 a, data points represent the fatigue response of one specimen and data points with arrows represent those that did not fail within a prescribed number of cycles and the experiment was discontinued. Note that the control data is the same as that presented for young dentin in Fig. 2 a, with the addition of a few more points. A similar comparison of results for the flaw free control with specimens subjected to bur treatment and followed by etching with 37.5% gel for 15 s is shown in Fig. 3 b. Power law equations for the mean fatigue responses are presented in each diagram, along with the coefficient of determination (R 2 ) indicating the goodness of fit. According to the Mann Whitney U test, the fatigue strength of the treated control specimens receiving BT (Z = −5.6; p ≤ 0.0001) and BT + ET (Z = −5.5; p ≤ 0.0001) treatments were significantly lower than the flaw free control. However, there was no significant difference between the responses of the BT and the BT + ET groups (p > 0.05). The cutting and etching treatments increased the Ra from that resulting from the diamond slicing (0.2 μm) by nearly five times (≈1 μm). Although this increase had no effect on the static strength, the reductions in fatigue strength exceeded 30%.
The distributions in Fig. 3 highlight the additional value of using fatigue life diagrams to compare the resistance to fatigue failure. Not only do the BT and BT + ET treatments cause a significant reduction in fatigue strength, they also decrease the consistency in the fatigue life. The variability in fatigue data and relatively poor R 2 values of the power laws for the BT and BT + ET groups indicate that the experimental responses do not conform well to the average response defined by the power law models. This variation in fatigue strength is due to the flaws introduced at the surface during the cutting. As evident from the fatigue life distributions in Fig. 3 , the flaws caused by bur cutting were most severe and relatively inconsistent among the treated specimens. Clearly the fatigue strength of dentin is decreased by surface flaws that are introduced during the cavity preparation.
There are many challenges in the oral environment that may contribute to the fatigue behavior of dental materials and the hard tissue foundation. The acid production of biofilms results in the formation of secondary caries at the bonded interface between dentin and restorative materials, and serves as one of the primary causes of restored tooth failure . Yet, the potential synergism in fatigue caused by simultaneous cyclic loading and exposure to acidic conditions has received limited attention. Do et al. explored the influence of a reduction in pH from neutral (pH = 7) to lactic acidic (pH = 5) conditions to the fatigue properties of coronal dentin. The exposure to acidic conditions caused a significant reduction to the fatigue strength, as expected. The most interesting aspect of the investigation is that a significant degradation in fatigue strength developed after only 10 h of exposure to the acidic condition. The primary mechanism of degradation appeared to be subsurface porosity caused by demineralization, which results in loss of stiffness and corresponding increase in localized surface strains that accelerate the process of fatigue.
Changes to the microstructure of dentin caused by the acidic sections of biofilms and localized demineralization could degrade the mechanisms of toughening that are key to the fatigue crack growth resistance. That would reduce the durability of the tissue foundation supporting the restoration. Orrego et al. recently explored the influence of lactic acid exposure (pH = 5) on the fatigue crack growth resistance of human dentin for cracks extending within the mid-coronal regions. A comparison of the fatigue crack growth responses for mid-coronal dentin evaluated in the neutral and acid environments is shown in Fig. 4 a. As denoted from the shift of the fatigue crack growth responses to the upper left region of the diagram, the lactic acid environment caused a reduction in the fatigue crack growth resistance. The rate of cyclic crack extension for tissue exposed to the acidic solution is significantly greater (Z = −6.4665, p = 0.0005) than that within the neutral environment. Admittedly, there is limited influence of the environment on the stress intensity range necessary for initiation of cyclic crack extension. But when the two distributions are compared in terms of the rate of cyclic extension, the incremental growth rate increased by over a factor of 10 in the acidic condition. That corresponds to a reduction in the apparent fatigue life by a factor of 10!
The penetration of adhesive resins within the tubules and formation of tags could be envisioned as a form of reinforcement that also resists acid from penetrating within the lumens to access the crack. The importance of resin-adhesive penetration within the lumens on the fatigue crack growth response of mid-coronal dentin has also been explored. A comparison of the fatigue crack growth responses for dentin with open lumens and for dentin with lumens sealed with resin adhesive (Ultradent Products Inc., Peak Universal Bond) is shown in Fig. 4 b; both were subjected to acid exposure (pH = 5). As evident from the comparison of data in this diagram, sealing the lumens with resin adhesive did not affect the rate of cyclic crack growth significantly (Z = 0.4832, p = 0.629). Therefore, the movement of acid buffers within the lumens and/or sealing the lumens are not important to cyclic crack extension in dentin. The fatigue crack growth resistance is degraded under acidic conditions due to the exposure of acid within the open crack and its degradation of the principal mechanisms of toughening . Therefore, the exposure of dentin to acidic environments contributes to the development of caries, but it also increases the chance of tooth fractures via accelerated cyclic crack growth, and at lower mastication forces.
2
Background
There are many aspects of fatigue failures that are unique from those caused by static loading. The first and most important characteristic of fatigue is that failures occur at stresses that are generally much lower than the static “strength” as defined by a yield or ultimate strength. As such, cyclic loading facilitates failures that are not adequately predicted by measures of static strength. Secondly, fatigue is a stochastic process that is dependent on the growth and coalescence of intrinsic flaws in the material. That indicates that the fatigue strength is not deterministic because the mechanical response is largely dependent on conditions or processes that influence the internal flaw distribution and flaw sizes. Through the energetics of the cyclic loading process, the flaws grow to a size that reduces the ability to bear load. The third quality is that fatigue of a structure is more sensitive to changes in the surface quality than other aspects of mechanical behavior. And the fourth aspect of importance is that the fatigue resistance of a material is more likely to be influenced by other contributing forms of degradation (synergistic effects). For example, corrosion of metals is a well-known surface phenomenon that can reduce the fatigue strength and together they operate to accelerate the process of degradation .
These special qualities of fatigue are highly relevant to the success of restorations. Cutting of the preparation and the additional steps including etching, application of a primer, etc. contribute to both the surface quality and surface integrity of the materials serving as the foundation for adhesive bonds. In addition, the structure of these bonds is complex. Bonding to dentin and enamel involves at least three distinct materials, including the restoration, the resin adhesive and the hard tissue, as well as the interfaces between them. The difficulties realized in the placement and potential curing of the restorative materials increases the likelihood of introducing defects and their average size. Depending on the preparation and the mechanisms enrolled in anchoring the restoration, there are hybrid layers and interdiffusion zones with their own unique microstructure and properties. Consequently, the fatigue strength of the adhesive bond is controlled by all of these contributing elements. Identifying the weakest link is critical to the design and development of improved materials and practices that will extend the longevity of restorations.
Considering that the magnitude of cyclic stress borne by the forces of mastication are small enough to avoid bulk fracture, then there are two potential modes of fatigue failure. The first mode is where failure originates from existing inherent defects in the material ( Fig. 1 a). These defects coalesce with cyclic loading and facilitate fracture through a reduction in capacity to bear load. This process is regarded as “stress-life fatigue” and is the most common mode of fatigue. Alternatively, fatigue failures can originate from a well-defined flaw or crack ( Fig. 1 b) that undergoes extension via cyclic loading until it reaches a length that permits fracture due to the magnitude of the stress intensity. This process is regarded as fatigue crack growth. Both of these modes of fatigue are relevant to dental materials and the practice of restorative dentistry. Contact fatigue and dynamic fatigue (or slow crack growth) are also important, primarily relevant to crown materials, and warrant separate treatments.
2.1
Stress-life fatigue
The stress-life fatigue behavior of a material is evaluated by subjecting specimens with well-defined geometry to cyclic loads that result in a desired magnitude of cyclic stress. While standards are widely available that guide the choice of specimen geometry and size for most engineered materials, there are limited testing standards for dental materials. Cyclic loading of the specimens is conducted via tension or flexure at an acceptable frequency, and the number of cycles to failure is documented. Flexure is often preferred as it generates a simple stress state and reduces the problems associated with gripping and alignment of specimens that is associated with tensile loading.
If the goal of fatigue testing is to understand the average stress that results in a specific finite “life”, e.g. 50k cycles to failure, then the applied load is adjusted in subsequent tests according to the staircase method to distinguish the corresponding stress amplitude that results in the life of question. However, if the goal is to understand the fatigue strength distribution over a range in stress that results from oral function, then a fatigue life diagram is developed. Fatigue life diagrams describing the fatigue strength distribution of human coronal dentin for two donor age groups are shown in Fig. 2 a. One benefit of constructing the fatigue life diagram is that the data can be modeled with a simple power law model ( Fig. 2 a), which describes the fatigue strength distribution over the entire range of finite life. The diagram also helps distinguish if the material exhibits a fatigue limit (S e , also termed endurance limit), which is the cyclic stress amplitude below which the material exhibits a tremendously long life (does not fail).
Considering that the average number of masticatory cycles ranges between one and two thousand per day , the fatigue life diagram and determination of the endurance limit should be customary for dental materials, despite requiring a greater investment of time. For example, in comparing the fatigue strength distributions of young and old dentin in Fig. 2 a, a comparison at a life of 1k cycles shows that the fatigue strength of the old dentin is roughly 25% less than that of young dentin. That comparison can be performed for any finite life, and as evident from the distribution in Fig. 2 a, the relative fatigue strength of old dentin decreases with longer definition of life. A comparison of the fatigue limits (S e ) indicates that the fatigue strength of old dentin is nearly 50% lower than that of young dentin . If the goal of restorative dentistry is to promote life-long oral health, then the comparisons using the endurance limit are most relevant. They are also most objective. Finite life comparisons are valuable as well, but they provide an incomplete picture, and sometimes even misleading view, of the fatigue response.
2.2
Fatigue crack growth
The fatigue crack growth resistance of a material is determined by subjecting specimens with well-defined geometry and a sharp crack to cyclic loads that results in incremental crack extension. There are no testing standards explicitly for dental materials that guide the choice of specimen geometry and size to use for fatigue crack growth evaluations. A number of different specimens have been used for this purpose . Fatigue crack growth evaluations on dental materials are often conducted using beams loaded in flexure or Compact Tension (CT) specimens , to generate cyclic tensile stresses that achieve an adequate stress intensity range for cyclic extension of the crack. Cyclic loading of the specimens is conducted at an acceptable frequency and the crack length is measured after specific intervals of loading. The history of crack extension is then evaluated using a fatigue crack growth diagram, where the average growth rate (Δa/ΔN) is plotted in terms of the average stress intensity range (ΔK) over the increments of cyclic extension.
Similar to the comparison of fatigue strength distributions in Fig. 2 a, a fatigue crack growth diagram for young and old dentin human coronal dentin is shown in Fig. 2 b. This data was obtained for cyclic crack growth perpendicular to the dentin tubules ; the definition of the two age groups is consistent with that used for the stress-life responses ( Fig. 2 a). The cyclic crack growth history within a single specimen of young coronal dentin (Young*) is highlighted to enable observation of how cyclic crack extension progresses. Although the growth response of each specimen can be quantified in terms of the classical initiation and steady-state growth behavior , they are not really necessary to show the utility of this approach. Details of quantitative treatments applied to biomaterials are available elsewhere .
The fatigue crack growth diagram in Fig. 2 b presents the responses for specimens obtained from the teeth of many young and old donors. The distribution of this experimental data shows that even within the young and old age groups that there is considerable variation in the responses. This variation is largely related to the spatial variations in microstructure of coronal dentin . Fatigue crack growth diagrams are useful as they enable simple comparisons of the resistance to cyclic crack growth. Responses to the lower right in this diagram signify a material with higher resistance to fatigue crack growth, while those to the upper left undergo fatigue crack growth more easily, as highlighted with “+” and “−” symbols in Fig. 2 b. It is clear from this general description that old dentin exhibits substantially lower fatigue crack growth resistance than that of young dentin. It is also evident that the initiation of fatigue crack growth from existing cracks starts at much lower stress intensity range in old dentin. A comparison of the average growth rates can be made as a function of the stress intensity range. Choosing a value of ΔK that is within the range of that generated by cyclic mastication , it is possible to compare the average steady-state cyclic crack growth rates, which is shown in Fig. 2 b for ΔK ≈ 0.75 MPa*m 0.5 . The ratio of the cyclic growth rates at this level of stress intensity for old and young dentin exceeds 100, indicating that cracks in old dentin grow at 100 times the rate of those in young tissue. According to the reciprocal of this value, the corresponding “life” of old teeth with cracks subjected to the same level of mastication would be less than 1% of the life of a young tooth. This simple comparison shows that substantial degradation that can occur from the introduction of cracks within teeth during restorative processes, and that this damage is much more detrimental with increasing patient age.
2.3
Some relevant examples
With this basic understanding of how to test and characterize the fatigue and fatigue crack growth behavior of materials, it is possible to address some factors relevant to dental practice. The first concern is the importance of introducing the cavity preparation and surface quality. As previously highlighted, fatigue responses are sensitive to the surface quality of materials. Finishing of the margins and polishing of the occlusal surface are considered important steps in the placement of direct restorations. But are these steps really important to the strength and longevity of the restoration?
Lohbauer et al. reported that surface texture is important to the strength of resin composites and glass ceramics, even under monotonic loading. For surfaces with average roughness (Ra) larger than 2.1 μm, flaws created by the surface treatments were large enough to have a significant effect on the strength. In regards to cutting the cavity, Staninec et al. showed that laser preparations introduced cracks in dentin that exceeded 100 μm in length under some treatment conditions and caused a significant reduction in strength. But the static strength of a material is generally less sensitive to surface condition than the fatigue strength. Indeed, Majd et al. reported that while there was no influence of burs or airjet surface treatments on the strength of dentin under quasi-static loading, both preparations caused significant reductions to the fatigue strength.
Cutting the preparation and etching are integral steps in the placement of resin composite restorations. Lee et al. evaluated the effects of cutting and etching on both the static and fatigue strengths of coronal dentin. The average flexure strength of coronal dentin specimens prepared using diamond abrasive slicing equipment (control) was 154 ± 24 MPa. It was found that there was no difference (p > 0.05) in the strength between these “flaw-free” controls and specimens prepared using bur treatment or etching treatments. Hence, there were no effects of bur cutting or etching to the static strength of dentin.
A comparison of the fatigue life distribution for the control specimens (prepared by diamond abrasive slicing) with specimens that were prepared using the bur treatment (BT) is shown in Fig. 3 a. Similar to the fatigue life diagram in Fig. 2 a, data points represent the fatigue response of one specimen and data points with arrows represent those that did not fail within a prescribed number of cycles and the experiment was discontinued. Note that the control data is the same as that presented for young dentin in Fig. 2 a, with the addition of a few more points. A similar comparison of results for the flaw free control with specimens subjected to bur treatment and followed by etching with 37.5% gel for 15 s is shown in Fig. 3 b. Power law equations for the mean fatigue responses are presented in each diagram, along with the coefficient of determination (R 2 ) indicating the goodness of fit. According to the Mann Whitney U test, the fatigue strength of the treated control specimens receiving BT (Z = −5.6; p ≤ 0.0001) and BT + ET (Z = −5.5; p ≤ 0.0001) treatments were significantly lower than the flaw free control. However, there was no significant difference between the responses of the BT and the BT + ET groups (p > 0.05). The cutting and etching treatments increased the Ra from that resulting from the diamond slicing (0.2 μm) by nearly five times (≈1 μm). Although this increase had no effect on the static strength, the reductions in fatigue strength exceeded 30%.
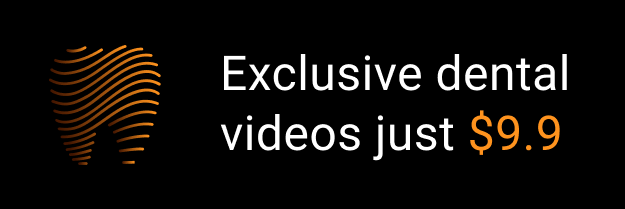