Abstract
There are concerns regarding the longevity of resin composite restorations and the clinical relevance of in vitro bond strength testing to the durability of dentin bonds in vivo.
Objective
The objectives of this investigation were to: (1) develop a new method of experimental evaluation for quantifying the durability of dentin bonds, (2) apply this method to characterize the interfacial strength of a selected commercial system under both monotonic and cyclic loading, and (3) distinguish mechanisms contributing to the interface degradation and failure.
Methods
A new method for fatigue testing the resin–dentin interface was developed based on a four-point flexure arrangement that includes two identical bonded interfaces. Cyclic loading of specimens comprised of coronal dentin bonded to a commercial resin composite and controls of resin composite was performed to failure within a hydrated environment. Scanning electron microscopy and nanoscopic dynamic mechanical analysis were used to evaluate failure mechanisms.
Results
The fatigue strength of the resin–dentin interface was significantly lower ( p ≤ 0.0001) than that of the resin composite and reported for dentin over the entire finite life regime. Defined at 1 × 10 7 cycles, the apparent endurance limit of the resin–dentin interface was 13 MPa, in comparison to 48 MPa and 44 MPa for the resin composite and dentin, respectively. The ratio of fully reversed endurance limit to ultimate strength of the interface (0.26) was the lowest of the three materials.
Significance
The proposed approach for characterizing the fatigue strength of resin–dentin bonds may offer new insights concerning durability of the bonded interface.
1
Introduction
Resin composites have become the most common material for direct posterior restorations . Composites offer benefits over amalgam including aesthetics and more conservative preparation size, but there are concerns regarding their longevity . The average clinical service life of bonded composite restorations is limited . Secondary caries, degradation of the restorative margins and fracture of the restoration are the most common forms of failure, with secondary caries being the primary cause of failure overall . As these failures appear to be largely related to the bond integrity or result from poor initial adhesion, the interface strength has emerged as the key performance metric.
Bond strength testing of restorative materials to dentin has been used to quantify the quality of adhesion since the invention of acid etching . Micro-tensile and shear tests quickly became the primary approaches for evaluating the success of new materials and to assess the influence of bonding procedures to clinical performance . Several variations of these two approaches are now used, from macroscopic methods with clinically relevant dimensions, to microscopic methods where the bonded surface area is well below 1 square millimeter (e.g. ). The dental materials community has adopted quasi-static tensile testing of the interface to infer performance in the oral environment, i.e. with high strength indicative of longevity. However, the applicability of these methods is being questioned, with clinical relevance being the primary concern .
In recognition that there are shortcomings to the current testing methods, the community is now addressing their potential weaknesses (e.g. ). Improvement in testing methods and specimen preparation procedures may help foster new understanding of the mechanical behavior of the resin–dentin interface. Yet, others have called for the development of new test methods that will better represent oral challenges and provide researchers with tools to make advances in resin adhesion to dentin. A load-bearing restoration bonded to oral hard tissues has to resist damage over many years. Indeed, occlusal function has been identified as a significant factor to the age of restorations at failure . Such an influence might simply be due to fatigue of the interface where cyclic stresses transmitted across the adhesive and hybrid layers accelerate degradation of the interface . In comparison to evaluations of monotonic strength, fatigue properties of the resin–dentin interface and their clinical relevance have received far less attention . Fatigue testing the interface could provide clinically relevant insight on dentin bond durability.
The present study introduces a novel approach for evaluating the strength of resin–dentin bonds under both monotonic and cyclic loading. The overall objective of this paper is to describe the merits of its application and supporting techniques for examining the degradation and failure of resin–dentin bonds as a result of fatigue. Here we present results for the fatigue strength achieved in dentin bonding with a specific commercial material, compare these results to the fatigue strength of dentin and resin composite, and discuss some of the primary contributors to fatigue failure of the interface.
2
Materials and methods
The investigation was aimed at developing a method for evaluating the fatigue strength of the resin–dentin bonded interface and validating the loading configuration using a finite element analysis. Thereafter, the newly developed method of evaluation was enrolled to assess the fatigue strength of the resin–dentin interface acheived using a commercial bonding system.
Bonded interface fatigue specimens were developed and involved coronal dentin, which was obtained from caries-free human second and third molars. The extracted molars were obtained with record of patient age (18 ≤ age ≤ 30) and gender from participating clinics within Maryland according to a protocol approved by the University of Maryland Baltimore County (#Y04DA23151). After extraction, the teeth were stored in Hank’s Balanced Salt Solution (HBSS) for less than 1 month, and then sectioned using a computer-controlled grinder (Chevalier Smart-H818II, Chevalier Machinery, Santa Fe Springs, CA, USA) with diamond abrasive slicing wheels (#320 mesh abrasives) and water spray coolant. All teeth were sectioned axially to obtain a slice of 2 mm thickness. Secondary sections were performed mesio-distally to obtain rectangular beams from the mid-coronal dentin with nominal dimensions of 2 mm × 2 mm × 10 mm ( Fig. 1 a). The dentin tubules of these beams were aligned perpendicular to their length ( Fig. 1 b), which is consistent with selected regions of the interface created by introducing a Class 2 cavity on a molar ( Fig. 2 a and b). After performing secondary sections, primer and adhesive (Clearfil SE Bond, Lot 062127, Kuraray America, Houston, TX, USA) were applied to the two opposing surfaces of each beam according to the manufacturer’s recommendations. Then the beams were placed within a dedicated mold ( Fig. 1 c) such that the approximal surfaces were oriented with tubules parallel to the bonding interface. Restorative resin composite (Clearfil AP-X, A2 color, Lot 1136AA; Kuraray America) was applied incrementally from the dentin beam surface and distributed as necessary to fill the mold cavities on each side of the dentin beam. The composite was cured on both sides for 40 s using a quartz–tungsten–halogen light-curing unit (Demetron VCL 401, Demetron, CA, USA) with output intensity of 600 mW/cm 2 and with tip diameter wider than 10 mm. The sections of bonded resin composite and dentin were released from the mold and sectioned using the slicer/grinder to obtain specimens with dimensions of roughly 2 mm × 2 mm × 12 mm ( Fig. 1 d). The specimens were examined for defects using an optical microscope and each side was polished lightly with hydration using #600 mesh emory paper. The specimens were stored in HBSS at room temperature (22 °C) for 24 h prior to further evaluation. A control group of resin composite specimens was prepared using the same approach, including molding, sectioning and polishing.
The prepared specimens were loaded to failure in either quasi-static or cyclic four-point flexure ( Fig. 2 a) using a universal testing system (EnduraTEC Model ELF 3200, Minnetonka, MN, USA), which has a load capacity and sensitivity of 225 N and ± 0.01 N, respectively. Quasi-static loading was applied with the specimens maintained in HBSS at room temperature using displacement control feedback at a crosshead rate of 0.06 mm/min. The instantaneous load and load-line displacement were monitored throughout loading at a frequency of 4 Hz. The flexural strength of the beams was determined using conventional beam theory in terms of the maximum measured load ( P ) and beam geometry (width b , thickness h ) according to 3Pl/bh 2 , where l is the distance from interior and exterior supports ( l = 3 mm). Ten specimens of the interface ( N = 10) and the resin composite control ( N = 10) were evaluated using this approach. The flexure strengths were compared using a one-way ANOVA and Tukey’s HSD post hoc analysis with the critical value (alpha) set at 0.05.
Cyclic loading of the bonded interface and control specimens was conducted using the same flexure configuration ( Fig. 2 a) under load control with frequency of 4 Hz and stress ratio ( R = ratio of minimum to maximum cyclic load) of 0.1. Fatigue testing was initiated using a maximum cyclic stress of approximately 90% of the flexural strength identified from the quasi-static experiments. For successive specimens, the maximum cyclic load was decreased in increments ranging from 5 to 15 MPa according to the staircase method of evaluation . The process continued until reaching the flexure stress amplitude at which specimens did not fail within 1.2 × 10 6 cycles. The stress-life ( S – N ) fatigue distribution was evaluated by plotting the cyclic stress amplitude against the number of cycles to failure. The fatigue life distribution of the specimens in each group that underwent fatigue failure was fitted using non-linear regression with a Basquin-type model of the form
σ = A ( N ) B
where A and B are the fatigue-life coefficient and exponent, respectively. These constants were obtained from a power law regression of the fatigue responses. The apparent endurance limit was estimated from the models for a fatigue limit defined at 1 × 10 7 cycles. The fatigue life distributions were compared using the Wilcoxon Sum Rank Test with p ≤ 0.05 considered significant. A total of 40 specimens were evaluated using this approach including the bonded interface specimens ( N = 20) and resin composite control ( N = 20).
Due to the presence of an interface in the resin–dentin beams, it was necessary to evaluate the stress distribution to confirm that beam theory provided a reliable measure of the flexural stress. Thus, a two-dimensional finite element analysis was performed using commercial software (ABAQUS 6.7-3; Dassault Systèmes Americas Corp., Waltham, MA, USA). Though not required, a full model was developed for the beam to simulate the resulting stress and strain distribution. The model beam was defined having three regions ( Fig. 3 a), i.e. the resin composite, resin adhesive and dentin, and meshed with approximately 3600 nodes and 1200 type CPE4 elements. For convenience the materials were treated as linear elastic with elastic modulus ( E ) and Poisson’s ratio ( ν ) defined for dentin ( E = 15 GPa, ν = 0.29) , resin composite (6.0 GPa, 0.26) [AP-X, Kuraray USA] and resin adhesive (4.4 GPa, 0.24) . Due to the limited information for the macroscopic elastic modulus of resin-infiltrated dentin, the hybrid layer and resin adhesive were combined and considered to have the same elastic properties (4.4 GPa, 0.24). It was recognized that viscoelastic deformation of the specimens may occur as a result of the non-zero mean stress generated by the fatigue loading protocol and duration of testing (0–3.5 days). However, the degree of viscoelastic deformation in each loading cycle was assumed to be small, which maintains applicability of the linear elastic model and its solution for the stress distribution. The beam was subjected to flexural loading according to the experimental configuration in Fig. 2 a through contact loading. The pins were defined as rigid body shells with frictionless contact between the pins and beam surfaces.
The flexure specimen geometry adopted for evaluating the resin–dentin bonding was defined with twin interfaces, akin to that of the double-notched beam configuration commonly used for evaluating engineering and natural materials . This configuration offers a special advantage in that both interfaces are subjected to a constant and equivalent bending moment. Symmetrical loading of the specimen was confirmed by using an articulating paper between the specimen and the loading pins, which ensured equi-distributed loading of the beam and equivalent normal stresses at each interface. As such, both interfaces were subjected to an equivalent macroscopic normal stress distribution during the loading history.
In both monotonic and cyclic loading one of the interfaces undergoes failure and the second interface, which has experienced the same stress history, effectively “freezes” the status of the microstructure at that moment. Though differences in the population and severity of defects can cause one of the two interfaces to undergo failure preferentially, the second interface survives and enables further evaluation. Here the specimens were evaluated using Scanning Electron Microscopy (SEM) and nanoscopic Dynamic Mechanical Analysis (nanoDMA) to assess the mechanisms of interface degradation caused by cyclic loading.
The fracture surfaces of selected specimens were inspected using a Scanning Electron Microscope (SEM: JEOL Model JSM-5600, Peabody MA, USA) in secondary electron imaging (SEI) mode. Prior to this analysis the specimens were dehydrated in an ascending ethanol series (70–100%), fixed in Hexamethyldisilazane, polished lightly using #600 mesh emory cloth and then sputtered with gold/palladium to enhance conductance of the dentin and resin adhesive. The fracture surfaces were inspected to distinguish differences between static and fatigue loading and to identify the origins of failure. It is important to note that due to the required dehydration of the specimens, the surviving interface dimensions were not evaluated using the SEM. Dehydration causes dimensional changes in the adhesive, and requires a relatively long period of time between completion of the testing and SEM evaluation. That enabled relaxation of the specimens and time to undergo further dimensional changes, which were not associated with the fatigue process.
To support nanoDMA evaluation of the interface that did not undergo failure, the portion of specimen containing the surviving resin–dentin interface was cold-mounted in Epofix epoxy resin (Struers, Cleveland, OH, USA). The side of the specimen was exposed, thereby revealing both the tension and compression areas of the specimen. Polishing was performed with diamond particle suspensions (Buehler) of sizes 9, 3, and 0.04 μm to produce a highly polished surface with a roughness of less than 50 nm RMS. Both mounting and polishing of the specimens were performed with hydration. NanoDMA was performed with a Triboindenter (Model TI 900, Hysitron, Minneapolis, MN, USA) and a Berkovich diamond indenter with a 100-nm tip radius. Scanning mode dynamic loading was conducted over scan areas of 30 μm × 30 μm with 4 μN contact load, 2 μN dynamic loading amplitude and dynamic loading frequency of 100 Hz. The evaluation was performed with hydration using a layer of ethylene glycol over the specimen surface to prevent water evaporation . This is a critical difference between the SEM and nanoDMA analysis. In addition, application of nanoDMA enabled an assessment of changes in mechanical behavior of the interface resulting from fatigue. The contact load and displacement signals were used to calculate the phase angle and to generate maps of the complex ( E *) modulus distribution for the dentin, resin adhesive and hybrid zone and restorative resin. An unloaded interface specimen (control) was also evaluated using these techniques after immersion in HBSS for 24 h. Additional details regarding application of nanoDMA and evaluating the resin–dentin interface using this approach is described in .
2
Materials and methods
The investigation was aimed at developing a method for evaluating the fatigue strength of the resin–dentin bonded interface and validating the loading configuration using a finite element analysis. Thereafter, the newly developed method of evaluation was enrolled to assess the fatigue strength of the resin–dentin interface acheived using a commercial bonding system.
Bonded interface fatigue specimens were developed and involved coronal dentin, which was obtained from caries-free human second and third molars. The extracted molars were obtained with record of patient age (18 ≤ age ≤ 30) and gender from participating clinics within Maryland according to a protocol approved by the University of Maryland Baltimore County (#Y04DA23151). After extraction, the teeth were stored in Hank’s Balanced Salt Solution (HBSS) for less than 1 month, and then sectioned using a computer-controlled grinder (Chevalier Smart-H818II, Chevalier Machinery, Santa Fe Springs, CA, USA) with diamond abrasive slicing wheels (#320 mesh abrasives) and water spray coolant. All teeth were sectioned axially to obtain a slice of 2 mm thickness. Secondary sections were performed mesio-distally to obtain rectangular beams from the mid-coronal dentin with nominal dimensions of 2 mm × 2 mm × 10 mm ( Fig. 1 a). The dentin tubules of these beams were aligned perpendicular to their length ( Fig. 1 b), which is consistent with selected regions of the interface created by introducing a Class 2 cavity on a molar ( Fig. 2 a and b). After performing secondary sections, primer and adhesive (Clearfil SE Bond, Lot 062127, Kuraray America, Houston, TX, USA) were applied to the two opposing surfaces of each beam according to the manufacturer’s recommendations. Then the beams were placed within a dedicated mold ( Fig. 1 c) such that the approximal surfaces were oriented with tubules parallel to the bonding interface. Restorative resin composite (Clearfil AP-X, A2 color, Lot 1136AA; Kuraray America) was applied incrementally from the dentin beam surface and distributed as necessary to fill the mold cavities on each side of the dentin beam. The composite was cured on both sides for 40 s using a quartz–tungsten–halogen light-curing unit (Demetron VCL 401, Demetron, CA, USA) with output intensity of 600 mW/cm 2 and with tip diameter wider than 10 mm. The sections of bonded resin composite and dentin were released from the mold and sectioned using the slicer/grinder to obtain specimens with dimensions of roughly 2 mm × 2 mm × 12 mm ( Fig. 1 d). The specimens were examined for defects using an optical microscope and each side was polished lightly with hydration using #600 mesh emory paper. The specimens were stored in HBSS at room temperature (22 °C) for 24 h prior to further evaluation. A control group of resin composite specimens was prepared using the same approach, including molding, sectioning and polishing.
The prepared specimens were loaded to failure in either quasi-static or cyclic four-point flexure ( Fig. 2 a) using a universal testing system (EnduraTEC Model ELF 3200, Minnetonka, MN, USA), which has a load capacity and sensitivity of 225 N and ± 0.01 N, respectively. Quasi-static loading was applied with the specimens maintained in HBSS at room temperature using displacement control feedback at a crosshead rate of 0.06 mm/min. The instantaneous load and load-line displacement were monitored throughout loading at a frequency of 4 Hz. The flexural strength of the beams was determined using conventional beam theory in terms of the maximum measured load ( P ) and beam geometry (width b , thickness h ) according to 3Pl/bh 2 , where l is the distance from interior and exterior supports ( l = 3 mm). Ten specimens of the interface ( N = 10) and the resin composite control ( N = 10) were evaluated using this approach. The flexure strengths were compared using a one-way ANOVA and Tukey’s HSD post hoc analysis with the critical value (alpha) set at 0.05.
Cyclic loading of the bonded interface and control specimens was conducted using the same flexure configuration ( Fig. 2 a) under load control with frequency of 4 Hz and stress ratio ( R = ratio of minimum to maximum cyclic load) of 0.1. Fatigue testing was initiated using a maximum cyclic stress of approximately 90% of the flexural strength identified from the quasi-static experiments. For successive specimens, the maximum cyclic load was decreased in increments ranging from 5 to 15 MPa according to the staircase method of evaluation . The process continued until reaching the flexure stress amplitude at which specimens did not fail within 1.2 × 10 6 cycles. The stress-life ( S – N ) fatigue distribution was evaluated by plotting the cyclic stress amplitude against the number of cycles to failure. The fatigue life distribution of the specimens in each group that underwent fatigue failure was fitted using non-linear regression with a Basquin-type model of the form
σ = A ( N ) B
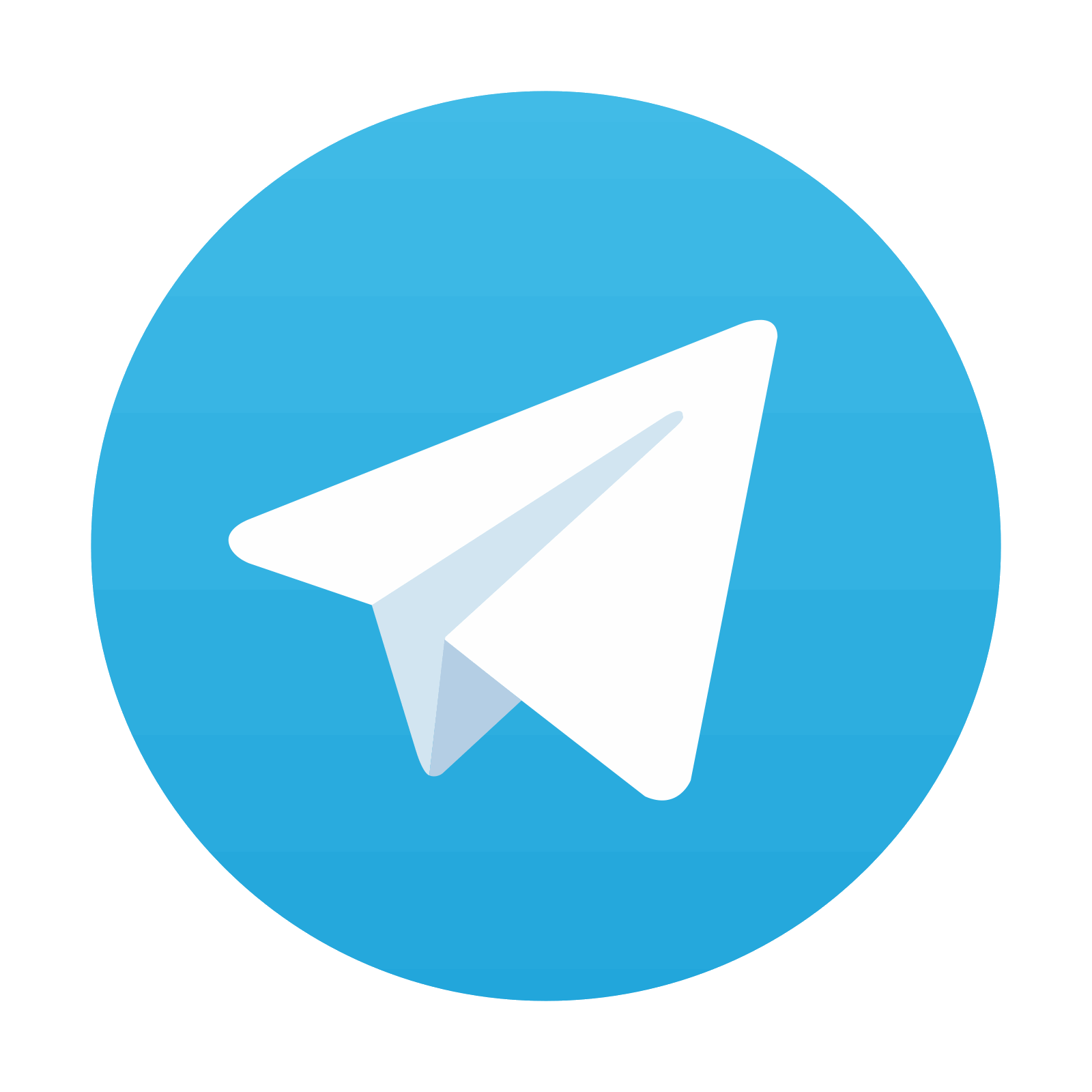
Stay updated, free dental videos. Join our Telegram channel

VIDEdental - Online dental courses
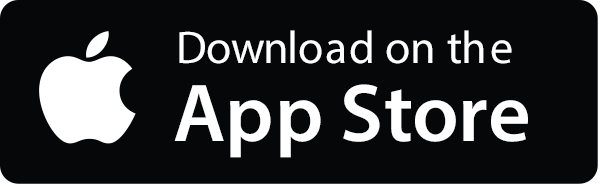
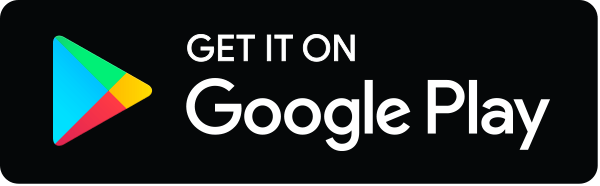