Highlights
- •
Synchrotron Radiation.
- •
Visualization of micromotion in implant–abutment connection.
Abstract
Objectives
Based on the current lack of data and understanding of the wear behavior of dental two-piece implants, this study aims for evaluating the microgap formation and wear pattern of different implants in the course of cyclic loading.
Methods
Several implant systems with different conical implant–abutment interfaces were purchased. The implants were first evaluated using synchrotron X-ray high-resolution radiography (SRX) and scanning electron microscopy (SEM). The implant–abutment assemblies were then subjected to cyclic loading at 98 N and their microgap was evaluated after 100,000, 200,000 and 1 million cycles using SRX, synchrotron micro-tomography (μCT). Wear mechanisms of the implant–abutment connection (IAC) after 200,000 cycles and 1 million cycles were further characterized using SEM.
Results
All implants exhibit a microgap between the implant and abutment prior to loading. The gap size increased with cyclic loading with its changes being significantly higher within the first 200,000 cycles. Wear was seen in all implants regardless of their interface design. The wear pattern comprised adhesive wear and fretting. Wear behavior changed when a different mounting medium was used (brass vs. polymer).
Significance
A micromotion of the abutment during cyclic loading can induce wear and wear particles in conical dental implant systems. This feature accompanied with the formation of a microgap at the IAC is highly relevant for the longevity of the implants.
1
Introduction
Two-piece dental titanium implants have been successfully used in dentistry for decades . In the oral cavity they have to resist dynamic forces and morse taper (conical) implant–abutment connections (IAC) have proven to be more fatigue resistant to mechanical loading . Nevertheless technical failures like veneer chipping or -fracture, screw- or abutment loosening as well as implant fractures have been reported amounting up to 30–41% within 5 years . Besides technical complications a variety of biological complications occur among which are periimplant mucositis and periimplantitis with a prevalence of 43% and 22% respectively . The etiopathogenesis of periimplantitis is not yet fully understood . Besides bacterial contamination wear at the implant–abutment interface with discharge of wear debris is currently discussed . The existence of titanium ions and particles in periimplantitis tissue has been documented . Micromotion of the abutment during loading has been described but its consequences have rarely been investigated in zirconia abutments . Cylic loading of zirconia abutments in titanium implants has proven to induce wear of the titanium implant in butt-joint and conical implant–abutment interfaces . Fatigue loading has also proven to increase the micromotion within the implant–abutment assembly using measurements of the microgap behavior with synchrotron-based X-rays (SRX) . SRX analysis feature higher spatial resolution and material contrast as compared to laboratory based X-rays. SRX and Synchrotron-based micro-tomography (μCT) allows for the detection of microgaps down to 0.1 μm and thus the deviation of the position of assembled metallic components . To date the influence of the micromotion within a conical IAC on the interface with regard to the wear behavior, microgap formation and abutment displacement has not been studied nor visualized yet.
The goal of this study was to analyze and visualize the microgap size after cyclic loading and the wear pattern with regard to the IAC-design.
2
Materials and method
2.1
Implant description and preparation
Within this study four implant systems (Nobel Active (NA), Nobel Biocare, Gothenburg, Sweden; Ankylos CX (AN), AstraDentsply, Mannheim, Germany; Bone Level (BL), Straumann, Waldenburg, Switzerland; Astra OsseoSpeed™ TX (AS), AstraDentsply) were used, each implant system being represented by 8 identical samples. Of these 32 samples implants, abutments and screws were purchased from the manufacturers. The three components implant, abutment and screw were assembled under a laminar flow using sterile gloves to minimize contamination of the IAC. They were then screw-tightened using the system-specific torque recommended by the manufacturers with calibrated tools. Details regarding the implants and the IAC-design are listed in Table 1 .
Implant data | Implant diameter/length (mm) | Cone angle (°) | Length of mating-zone (mm) |
---|---|---|---|
Ankylos C/X B11 Ref. 17-0554/31010430 | 4.5/11 | 5.7 | 1.84 |
OsseoSpeedTX Ref.24952 | 4.5/11 | 10.9 | 2.51 |
Nobel Active Internal RP Ref. 34132 | 4.3/11.5 a (4.0/11.5) | 11.7 | 0.85 |
Bone Level RC, SLA Ref 021.4412 | 4.1/12 | 15 | 0.72 |
For the dynamic loading in a computer-controlled dual axis-chewing simulator (Willytec, Munich, Germany), all implants were embedded to the implant shoulder in an autopolymerizing acrylic resin (Technovit ® 4000, Heraeus Kulzer, Wehrheim, Germany) into special sample holders by the use of silicon indices (Twinduo; Picodent, Wipperfuerth, Germany; Shore A hardness: 90) as described previously at an angle of 30˚ to the vertical axis ( Fig. 1 ). The resin had a Young’s modulus of approximately 2–2.2 GPa.
After the each one of the first two loading regimens (100,000 and 200,000 cycles) the specimens were removed from the resin and embedded in a brass cylinder according to DIN EN ISO 14801:2007 for the synchrotron evaluation ( Fig. 1 ).
After 200,000 cycles the implants remained in the brass cylinder for further cyclic loading. The workflow of the implants comprised the procedures depicted in Fig. 2 .
To ensure that the direction of force application remains known during the experiment the specimens were marked with a permanent marker on the outer implant body surface. At 200,000 cycles 4 implants (one implant of each implant system) were disassembled after synchrotron examination and prepared for scanning electron microscopy (SEM) and EDX analysis.
2.2
Dynamic loading
The chewing simulator-environment consisted of eight identical sample chambers and a stepper motor controlling vertical movements of the antagonists (Steatit ® ceramic balls, 6 mm in diameter, Hoechst Ceram Tec, Wunsiedel, Germany) against the implant samples. The applied load in the chewing simulator was 98 N (10 kg). The cyclic loading was set at 2 Hz. During the dynamic loading, all samples were examined twice a day.
Loading of each specimen was performed for 100,000 cycles and a further 100,000 and 800,000 cycles in the testing machine. 27 implants of the 32 implants were loaded for 1 million cycles, 4 out of the 5 remaining implants were examined in SEM after 200,000 cycles.
2.3
Synchrotron evaluation
2.3.1
Radiography
In order to evaluate their initial state prior to loading all samples were inspected by SRX after the assembly of the implant–abutment complex. The initial microgaps were calculated from this data using a procedure that has been described previously . The measurements of the microgap were performed at the upper IAC (ROI 1) opposite to the side of force application and in the lower IAC (ROI 2) at the side of force application ( Fig. 3 ), as it was previously described to be the area of highest changes after cyclic loading ( Fig. 4 ).
2.3.2
Computed tomography and radiography
After 100,000, 200,000 and 1 million cycles a synchrotron μCT was performed as described previously .
The beam times at the initial state, after 100.000 and 1 million cycles have been taking place at the European Synchrotron Radiation Facility (ESRF) in Grenoble (France) and after 200.000 cycles at the Berliner Elektronenspeicherring-Gesellschaft für Synchrotronstrahlung m.b.H. (BESSY) in Berlin (Germany).
For the radiographic measurements of gap size at IAC a comparison between on the one hand simulated forward and back-projection and on the other hand measured gray values are performed. In detail the shape of the phase contrast fringes of a comparable model of the IAC is simulated. This is compared with the ascertained gray value pathway perpendicular to the IAC. By fitting the gap size in the model for the simulation, the gap size is identified.
For tomographic measurements a more complex method is used. For good overview of the IAC gap size distribution a special illustration is used, as described by using the gap simulation of , it becomes easily clear, that for small gap sizes (<30 μm) the standard deviation of the phase contrast images is linear dependent to gap size. Under this assumption the gap size determination is performed in the reconstructed volume. In order to enhance comparability with the Radiography measurements after assembly only two regions of interest were examined.
2.4
SEM and EDX
Before assembly and after 200,000 cycles as well as after 1 million cycles the internal interface of the respective implants were scanned using a scanning electron microscope (FEI Quanta 250 FEG) with a magnification of 50× to 3000× (magnification is the ratio of the size of the image on a 19 in. monitor to the size of the scanned area). EDX Analysis was performed using Oxford INCA x-act detector and INCA software.
2.5
Statistical evaluation
The differences of the microgap size regarding the loading cycles and the implant systems were analyzed using the two-factorial nonparametric analysis. The analysis was performed using SAS 9.1 (SAS Institute Inc., Cary, NC, USA). A p -value <0.05 was considered statistically significant.
2
Materials and method
2.1
Implant description and preparation
Within this study four implant systems (Nobel Active (NA), Nobel Biocare, Gothenburg, Sweden; Ankylos CX (AN), AstraDentsply, Mannheim, Germany; Bone Level (BL), Straumann, Waldenburg, Switzerland; Astra OsseoSpeed™ TX (AS), AstraDentsply) were used, each implant system being represented by 8 identical samples. Of these 32 samples implants, abutments and screws were purchased from the manufacturers. The three components implant, abutment and screw were assembled under a laminar flow using sterile gloves to minimize contamination of the IAC. They were then screw-tightened using the system-specific torque recommended by the manufacturers with calibrated tools. Details regarding the implants and the IAC-design are listed in Table 1 .
Implant data | Implant diameter/length (mm) | Cone angle (°) | Length of mating-zone (mm) |
---|---|---|---|
Ankylos C/X B11 Ref. 17-0554/31010430 | 4.5/11 | 5.7 | 1.84 |
OsseoSpeedTX Ref.24952 | 4.5/11 | 10.9 | 2.51 |
Nobel Active Internal RP Ref. 34132 | 4.3/11.5 a (4.0/11.5) | 11.7 | 0.85 |
Bone Level RC, SLA Ref 021.4412 | 4.1/12 | 15 | 0.72 |
For the dynamic loading in a computer-controlled dual axis-chewing simulator (Willytec, Munich, Germany), all implants were embedded to the implant shoulder in an autopolymerizing acrylic resin (Technovit ® 4000, Heraeus Kulzer, Wehrheim, Germany) into special sample holders by the use of silicon indices (Twinduo; Picodent, Wipperfuerth, Germany; Shore A hardness: 90) as described previously at an angle of 30˚ to the vertical axis ( Fig. 1 ). The resin had a Young’s modulus of approximately 2–2.2 GPa.
After the each one of the first two loading regimens (100,000 and 200,000 cycles) the specimens were removed from the resin and embedded in a brass cylinder according to DIN EN ISO 14801:2007 for the synchrotron evaluation ( Fig. 1 ).
After 200,000 cycles the implants remained in the brass cylinder for further cyclic loading. The workflow of the implants comprised the procedures depicted in Fig. 2 .
To ensure that the direction of force application remains known during the experiment the specimens were marked with a permanent marker on the outer implant body surface. At 200,000 cycles 4 implants (one implant of each implant system) were disassembled after synchrotron examination and prepared for scanning electron microscopy (SEM) and EDX analysis.
2.2
Dynamic loading
The chewing simulator-environment consisted of eight identical sample chambers and a stepper motor controlling vertical movements of the antagonists (Steatit ® ceramic balls, 6 mm in diameter, Hoechst Ceram Tec, Wunsiedel, Germany) against the implant samples. The applied load in the chewing simulator was 98 N (10 kg). The cyclic loading was set at 2 Hz. During the dynamic loading, all samples were examined twice a day.
Loading of each specimen was performed for 100,000 cycles and a further 100,000 and 800,000 cycles in the testing machine. 27 implants of the 32 implants were loaded for 1 million cycles, 4 out of the 5 remaining implants were examined in SEM after 200,000 cycles.
2.3
Synchrotron evaluation
2.3.1
Radiography
In order to evaluate their initial state prior to loading all samples were inspected by SRX after the assembly of the implant–abutment complex. The initial microgaps were calculated from this data using a procedure that has been described previously . The measurements of the microgap were performed at the upper IAC (ROI 1) opposite to the side of force application and in the lower IAC (ROI 2) at the side of force application ( Fig. 3 ), as it was previously described to be the area of highest changes after cyclic loading ( Fig. 4 ).
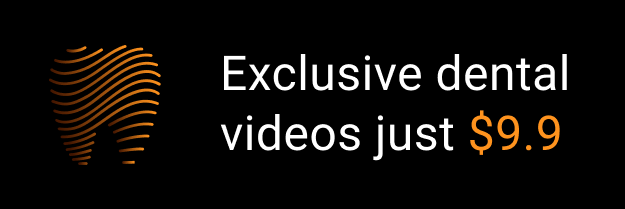