Abstract
Objective
The use of a 30 μm alumina–silica coated particle sand (CoJet™ Sand, 3M Espe), has shown to enhance the adhesion of resin cements to Y-TZP. The question is whether or not sandblasting 30 μm particles does negatively affect the fatigue limit ( S – N curves) and the cumulative survival of Y-TZP ceramics.
Method
Four zirconia materials tested were: Zeno (ZW) (Wieland), Everest ZS (KV) (KaVo), Lava white (LV) and Lava colored (LVB) (3M Espe). Fatigue testing ( S – N ) was performed on 66 bar of 3 mm × 5 mm × 40 mm with beveled edges for each zirconia material provided by the manufacturers. One half of the specimens were CoJet sandblasted in the middle of the tensile side on a surface of 5 mm × 6 mm. Cyclic fatigue ( N = 30/group) (sinusoidal loading/unloading at 10 Hz between 10% and 100% load) was performed in 3-point-bending in a water tank. Stress levels were lowered from the initial static value (average of N = 3) until surviving 1 million cycles. Fatigue limits were determined from trend lines. Kaplan–Meier survival analysis was performed to determine the failure stress at the median percentile survival level for 1 million of cycles before and after sandblasting. The statistical analyses used the log-rank test. Characterization of the critical flaw was performed by SEM for the majority of the failed specimens.
Results
The fatigue limits “as received” (ctr) were: LV = 720 MPa, LVB = 600 MPa, KV = 560 MPa, ZW = 470 MPa. The fatigue limits “after CoJet sandblasting” were: LV = 840 MPa, LVB = 788 MPa, KV = 645 MPa, ZW = 540 MPa. The increase in fatigue limit after sandblasting was 15% for Zeno (ZW) and Everest (KV), 17% for Lava (LV) and 31% for Lava colored (LVB). The KM median survival stresses in MPa were: ZW(ctr) = 549 (543–555), ZW(s) = 587 (545–629), KV(ctr) = 593 (579–607), KV(s) = 676 (655–697), LVB(ctr) = 635 (578–692), LVB(s) = 809 (787–831), LV(ctr) = 743 (729–757), LV(s) = 908 (840–976). Log-rank tests were significantly different ( p < 0.001) for all sandblasted groups vs. the “as received” except for Zeno (Wieland) ( p = 0.295). Failures started from both intrinsic and machined flaws.
Significance
30 μm particle sandblasting did significantly improve the fatigue behavior of three out of four Y-TZP ceramic materials and can therefore be recommended for adhesive cementation procedures. This study was supported in part by grants from the Swiss Society for Reconstructive Dentistry (SSRD) and 3M Espe.
1
Introduction
Due to its excellent mechanical properties (i.e. bending strength and toughness) Yttria stabilized tetragonal zirconia polycrystal (Y-TZP) has become an attractive high-toughness core material for fixed dental restorations . The innovation of zirconia ceramics lies in its stress-induced toughening mechanism, which impedes crack propagation . During stress application, the tetragonal crystallites ( t ) transform into their monoclinic ( m ) form at the crack tip. The concomitant 3–5% expansion in volume leads to the development of internal compressive stresses that oppose crack propagation and thus increase the material’s fracture toughness ( K Ic ) and strength. Transformation can be induced by any externally applied stress such as generated by grinding, upon impact or during outright fracture. Grinding Y-TZP and sandblasting will create compressive stresses in the surface due to the t → m phase transformation . The magnitude of the increase in strength depends on the volume % of transformed ZrO 2 and the depth of the compressive layer underneath the surface (i.e. the “transformed zone depth”) . Still, forceful grinding will introduce deep surface flaws, which extend beyond the surface compressive layer. These will act as stress concentrators and curtail the strength of the workpiece .
Sandblasting of zirconia ceramic with 50 or 110 μm alumina particles improves adhesion to resin-based cements due to increased surface roughness . Also, high adhesive bond strengths were demonstrated when sandblasting with 30 μm silica-coated alumina particles at ca. 2.8 bar in combination with phosphate monomer resin-based cements . Concerns have been raised regarding the surface damages after sandblasting with alumina particles of 50–120 μm at pressures of 2.8–4 bar . Kosmač reported a decline in the reliability (from Weibull m = 11–7.5) of zirconia specimens after sandblasting with 110 μm alumina particles. The deterioration was in direct relation with the damages affecting the ceramic surface. The authors warned that these impact flaws might develop into severe stress intensifiers when exposed to a wet environment under cyclic loading and thus facilitate crack nucleation, even at lower levels of applied stress. Along the same lines, Zhang et al. reported a 30% decrease in strength during contact fatigue testing after sandblasting mirror polished surfaces of Y-TZP with 50 μm alumina particles at 2.8 bar. The decline was explained by the formation of microcracks (>4 μm) in the specimens which under sustained loading would grow and promote radial crack initiation .
Clinically, the typical surfaces of zirconia frameworks for dental restoration are not mirror polished but utilized as machined by the CAD–CAM devices. They thus harbor a variety of initial surface flaws, which may be further affected by sandblasting. On CAD–CAM machined Lava™ zirconia specimens with Ra = 0.21 μm, Curtis et al. found no difference in biaxial strength and reliability when tested in water after sandblasting with 25, 50 or 110 μm Al 2 O 3 . In contrast Wang et al. demonstrated an increase in dry static flexural strength and reliability after sandblasting a CAD–CAM machined Y-TZP (Cercon ® ) (Ra = 1.9 μm) ceramic with 50 μm alumina particles. These seemingly contradictory findings may actually be related to a variety of factors such as the nature of the defects induced by machining and processing, the t – m phase distribution in the surface and the residual stress state prior to testing.
Concerns have been also expressed regarding the degradation of zirconia ceramic in the oral environment due to the exposure to water and mechanical stress over prolonged periods . Still, Y-TZP is currently the strongest ceramic in clinical use . Further, its sandblasting with 30 μm silica-coated alumina particles has been recommended to improve the adhesion to resin-based cements . It is unclear whether or not a “less aggressive” sandblasting with 30 μm silica-coated alumina particles does affect the fatigue resistance of CAD–CAM machined Y-TZP ceramics. In this context, stress vs. number of cycles diagrams ( S – N – or Wöhler curves) will allow the experimentalist to define a stress range (i.e. the “fatigue limit”) below which failure will not occur on any realistic time scale.
The objectives of the present study therefore were: (1) To draw S – N diagrams and determine the fatigue limits of four Y-TZP zirconia materials “as received” and “sandblasted” with 30 μm silica-coated alumina particles. Further to determine median Kaplan–Meier survival stresses under cyclic fatigue loading. (2) To characterize the type of flaws affecting fatigue failure using fractographic analysis. (3) To determine the proportions of tetragonal vs. monoclinic phases “as received” and “sandblasted” using X-ray diffraction.
2
Materials and methods
The zirconia materials used in this study are summarized in Table 1 . Bars 40 mm × 3 mm × 5 mm with beveled edges were provided “as received” by the manufacturers for each Y-TZP material. Prior to testing, each bar was subjected to four firing cycles (930 °C, 900 °C, 890 °C, 880 °C) corresponding to the firing programs for “liner”, “dentin 1”, “dentin 2” and “glaze” of a zirconia veneering ceramic (Zirox ® , Wieland) to duplicate the effect of veneering ceramic application.
Brand | Materials | Manufacturer |
---|---|---|
LAVA (LV) | 3Y-TZP | 3M Espe, Seefeld, D |
LAVA colored (LVB) | 3Y-TZP | 3M Espe, Seefeld, D |
EVEREST ZS (KV) | 3Y-TZP | KaVo Dental GmbH, Biberach, D |
ZENO Zr (ZW) | 3Y-TZP | Wieland, Dental, Pforzheim, D |
2.1
Material characterization
2.1.1
Microstructure, density, surface roughness (Ra)
The microstructure of each material was determined after thermal etching mirror polished surfaces. Thermal etching was performed 50 °C below the sintering temperature for 30 min. Scanning Electron Microscope images were taken at 20,000× magnification and the average grain size was determined using the linear intercept count method (ASTM E112-96(2004)e2).
The density of the sintered zirconia specimens was measured on N = 5 specimens per material (3 measurements per specimen) using Archimedes’ method.
The surface roughness ( N = 3/material) was measured on the “as received” bars using an electro-mechanical profilometer (M1, Mahr) equipped with a drive unit (PGK, Mahr) and a measuring sensor (MFW-250, Mahr) with a 40 nm z resolution. The bars were mounted on an XY cross table. As received and after sandblasting, areas of 5.6 mm × 1.75 mm oriented parallel to the long axis of the bars were scanned using a 2 μm radius diamond stylus at a speed of 0.5 mm/s and an applied force of 0.5 mN. Frames of 51 line profiles with y -axis steps of 60 μm were obtained. To determine the average surface roughness (Ra), a Gaussian profile filter (ISO 11562) with the cut-off wavelength set at λ c = 0.8 mm and an evaluation length of l n = 5.6 mm was used.
2.1.2
Weibull parameters
Weibull’s characteristic ( σ 0 ) strengths and moduli ( m ) were determined from 10 mirror polished (1 μm) bars of each material tested. To this effect, a customized 3-point bending device was mounted into a water tank ( Fig. 1 ). The lateral axes (rollers) supporting the specimens were incorporated in anti-torsion devices allowing rotation, thus compensating for minor unevennesses in the surfaces. The span between the rollers was set to 30 mm and the crosshead speed was 0.5 mm/min.

2.2
S – N diagrams, fatigue limits, survival analysis
Sixty-six CAD–CAM bars as machined by the manufacturers were used for fatigue testing. Half of the specimens were sandblasted in their midportion (i.e. at the maximum tensile stress) with 30 μm silica-coated alumina particles (CoJet™ Sand, 3M ESPE) on a surface of 6 mm × 5 mm for 20 s. Sandblasting was performed using an air abrasion gun (RondoFlex 2013, KaVo) at 2.5 bar with the tip inclined approximately 45° and at a distance of 7 mm. The specimens were then ultrasonically cleaned in pure ethanol for 10 min using a sonic device (Micro 10+, Unident) set to a low frequency range (28–34 kHz). Fatigue tests were conducted on a servo-hydraulic machine (Schenck PSB1017) using the 3-point bending assembly immersed in a water tank ( Fig. 1 ). The span between the rollers was set to 30 mm. The fatigue test conditions were as follows: (i) sinusoidal loading/unloading cycles at a frequency of 10 Hz, (ii) a stress ratio of R = σ min / σ max = 0.1 (amplitude of 90%) and (iii) at the onset of the test, a 5 s ramp (i.e. 50 cycles) before the maximum imposed stress level ( σ max ) was reached.
Prior to fatigue testing, the monotonic failure stresses were determined from 3 specimens of each material and surface conditions (i.e. “as received” vs. “sandblasted”). Testing was performed in water at a crosshead speed of 0.5 mm/min. From the average monotonic stresses recorded, the entry fatigue stress level was set 20% below the monotonic stress at failure. S – N diagrams (stress vs. number of cycles to failure) were generated from 30 specimens per material and per surface condition. Fatigue limits were set at one million cycles and determined from trend lines using a least square linear regression fit. The test was censored at 10 6 cycles and Kaplan–Meier survival analysis was performed to determine the failure stress at the median percentile. Kaplan–Meier analysis is a statistical method used to estimate the cumulative probability of survival for specimens or prostheses at each point in time, which is calculated as the product of conditional probability of survival for all previous time intervals . Kaplan–Meier analysis is useful in preventing probability of survival estimates from being biased by specimens or prostheses that survive beyond the window of observation , also known as right-censored data or run-out specimens.
2.3
Failure origins
The fracture surface of the first 20 failed specimens in each group was first examined under a stereo microscope at maximum 57× magnification and then investigated by scanning electron microscopy to determine the origin of the failure (i.e. intrinsic flaws due to processing or extrinsic flaws due to machining). Fractographic analysis was performed in accordance with the NIST Recommended practice guide for fractography of glasses and ceramics . Features such as mirrors and hackle were identified on the fracture surface and aided in locating the origin of the failure.
2.4
X-ray diffraction
X-ray diffraction (XRD) was employed to quantify the crystalline phases on the “as received” and after sandblasting each Y-TZP material. XRD analyses were conducted using a diffractometer (Siemens D5005, Bruker AXS) with Ni filtered Cu Kα radiation (1.5418 Å) at 35 mA and 40 kV. Diffraction data were collected from 26° to 65° 2 , with a step size of 0.010° and a scanning time of 2 s/step. A quantitative value of phase compositions of the “as received” and “after sandblasting” Y-TZP ceramics was obtained after applying Rietveld refinement analysis (Topas, Bruker AXS).
2
Materials and methods
The zirconia materials used in this study are summarized in Table 1 . Bars 40 mm × 3 mm × 5 mm with beveled edges were provided “as received” by the manufacturers for each Y-TZP material. Prior to testing, each bar was subjected to four firing cycles (930 °C, 900 °C, 890 °C, 880 °C) corresponding to the firing programs for “liner”, “dentin 1”, “dentin 2” and “glaze” of a zirconia veneering ceramic (Zirox ® , Wieland) to duplicate the effect of veneering ceramic application.
Brand | Materials | Manufacturer |
---|---|---|
LAVA (LV) | 3Y-TZP | 3M Espe, Seefeld, D |
LAVA colored (LVB) | 3Y-TZP | 3M Espe, Seefeld, D |
EVEREST ZS (KV) | 3Y-TZP | KaVo Dental GmbH, Biberach, D |
ZENO Zr (ZW) | 3Y-TZP | Wieland, Dental, Pforzheim, D |
2.1
Material characterization
2.1.1
Microstructure, density, surface roughness (Ra)
The microstructure of each material was determined after thermal etching mirror polished surfaces. Thermal etching was performed 50 °C below the sintering temperature for 30 min. Scanning Electron Microscope images were taken at 20,000× magnification and the average grain size was determined using the linear intercept count method (ASTM E112-96(2004)e2).
The density of the sintered zirconia specimens was measured on N = 5 specimens per material (3 measurements per specimen) using Archimedes’ method.
The surface roughness ( N = 3/material) was measured on the “as received” bars using an electro-mechanical profilometer (M1, Mahr) equipped with a drive unit (PGK, Mahr) and a measuring sensor (MFW-250, Mahr) with a 40 nm z resolution. The bars were mounted on an XY cross table. As received and after sandblasting, areas of 5.6 mm × 1.75 mm oriented parallel to the long axis of the bars were scanned using a 2 μm radius diamond stylus at a speed of 0.5 mm/s and an applied force of 0.5 mN. Frames of 51 line profiles with y -axis steps of 60 μm were obtained. To determine the average surface roughness (Ra), a Gaussian profile filter (ISO 11562) with the cut-off wavelength set at λ c = 0.8 mm and an evaluation length of l n = 5.6 mm was used.
2.1.2
Weibull parameters
Weibull’s characteristic ( σ 0 ) strengths and moduli ( m ) were determined from 10 mirror polished (1 μm) bars of each material tested. To this effect, a customized 3-point bending device was mounted into a water tank ( Fig. 1 ). The lateral axes (rollers) supporting the specimens were incorporated in anti-torsion devices allowing rotation, thus compensating for minor unevennesses in the surfaces. The span between the rollers was set to 30 mm and the crosshead speed was 0.5 mm/min.
2.2
S – N diagrams, fatigue limits, survival analysis
Sixty-six CAD–CAM bars as machined by the manufacturers were used for fatigue testing. Half of the specimens were sandblasted in their midportion (i.e. at the maximum tensile stress) with 30 μm silica-coated alumina particles (CoJet™ Sand, 3M ESPE) on a surface of 6 mm × 5 mm for 20 s. Sandblasting was performed using an air abrasion gun (RondoFlex 2013, KaVo) at 2.5 bar with the tip inclined approximately 45° and at a distance of 7 mm. The specimens were then ultrasonically cleaned in pure ethanol for 10 min using a sonic device (Micro 10+, Unident) set to a low frequency range (28–34 kHz). Fatigue tests were conducted on a servo-hydraulic machine (Schenck PSB1017) using the 3-point bending assembly immersed in a water tank ( Fig. 1 ). The span between the rollers was set to 30 mm. The fatigue test conditions were as follows: (i) sinusoidal loading/unloading cycles at a frequency of 10 Hz, (ii) a stress ratio of R = σ min / σ max = 0.1 (amplitude of 90%) and (iii) at the onset of the test, a 5 s ramp (i.e. 50 cycles) before the maximum imposed stress level ( σ max ) was reached.
Prior to fatigue testing, the monotonic failure stresses were determined from 3 specimens of each material and surface conditions (i.e. “as received” vs. “sandblasted”). Testing was performed in water at a crosshead speed of 0.5 mm/min. From the average monotonic stresses recorded, the entry fatigue stress level was set 20% below the monotonic stress at failure. S – N diagrams (stress vs. number of cycles to failure) were generated from 30 specimens per material and per surface condition. Fatigue limits were set at one million cycles and determined from trend lines using a least square linear regression fit. The test was censored at 10 6 cycles and Kaplan–Meier survival analysis was performed to determine the failure stress at the median percentile. Kaplan–Meier analysis is a statistical method used to estimate the cumulative probability of survival for specimens or prostheses at each point in time, which is calculated as the product of conditional probability of survival for all previous time intervals . Kaplan–Meier analysis is useful in preventing probability of survival estimates from being biased by specimens or prostheses that survive beyond the window of observation , also known as right-censored data or run-out specimens.
2.3
Failure origins
The fracture surface of the first 20 failed specimens in each group was first examined under a stereo microscope at maximum 57× magnification and then investigated by scanning electron microscopy to determine the origin of the failure (i.e. intrinsic flaws due to processing or extrinsic flaws due to machining). Fractographic analysis was performed in accordance with the NIST Recommended practice guide for fractography of glasses and ceramics . Features such as mirrors and hackle were identified on the fracture surface and aided in locating the origin of the failure.
2.4
X-ray diffraction
X-ray diffraction (XRD) was employed to quantify the crystalline phases on the “as received” and after sandblasting each Y-TZP material. XRD analyses were conducted using a diffractometer (Siemens D5005, Bruker AXS) with Ni filtered Cu Kα radiation (1.5418 Å) at 35 mA and 40 kV. Diffraction data were collected from 26° to 65° 2 , with a step size of 0.010° and a scanning time of 2 s/step. A quantitative value of phase compositions of the “as received” and “after sandblasting” Y-TZP ceramics was obtained after applying Rietveld refinement analysis (Topas, Bruker AXS).
3
Results
The materials characteristics regarding microstructure, density, roughness and Weibull parameters are summarized below.
3.1
Microstructure, density and roughness (Ra)
Fig. 2 displays the microstructure of the four Y-TZP materials at 20,000× magnification. The average grain size, standard deviation and range as determined by the “Linear interceptive count method” were as follows: Everest (KV) 383 nm (±47) (140–800 nm), Zeno (ZW) 383 nm (±47) (160–880 nm); Lava (LV) 537 nm (±47) (160–1200 nm); Lava colored (LVB) 643 nm (±61) (200–1600 nm).
Densities and roughness values are listed in Table 2 . The roughness of Everest (KV) “as received” specimens was the highest of the four materials because the machining grooves of these specimens were oriented in transverse direction while the other materials were ground longitudinally. With the exception of Everest (KV), the roughness slightly but significantly increased ( Table 2 ) after sandblasting with CoJet.
Zirconia groups | Density (g/cm 3 ) (SD) | Roughness (μm) (Ra) (SD) |
---|---|---|
Lava (LV) | 6.09 (0.02) | 0.23 (0.01) |
Lava sandblasted (LVs) | 0.26 (0.01) | |
Lava colored (LVB) | 6.12 (0.003) | 0.18 (0.01) |
Lava colored sandblasted (LVBs) | 0.27 (0.02) | |
Everest (KV) | 6.08 (0.01) | 0.75 (0.58) |
Everest sandblasted (KVs) | 0.71 (0.55) | |
Zeno (ZW) | 6.09 (0.004) | 0.30 (0.06) |
Zeno sandblasted (ZWs) | 0.35 (0.04) |
3.2
Weibull parameters
Weibull’s characteristic strength ( σ 0 ), moduli ( m ) and corresponding confidence intervals (CI) are summarized in Table 3 for the mirror polished bars. The characteristic strength of the Zeno ceramic was significantly inferior to the three others. No significant differences were evidenced in the moduli.
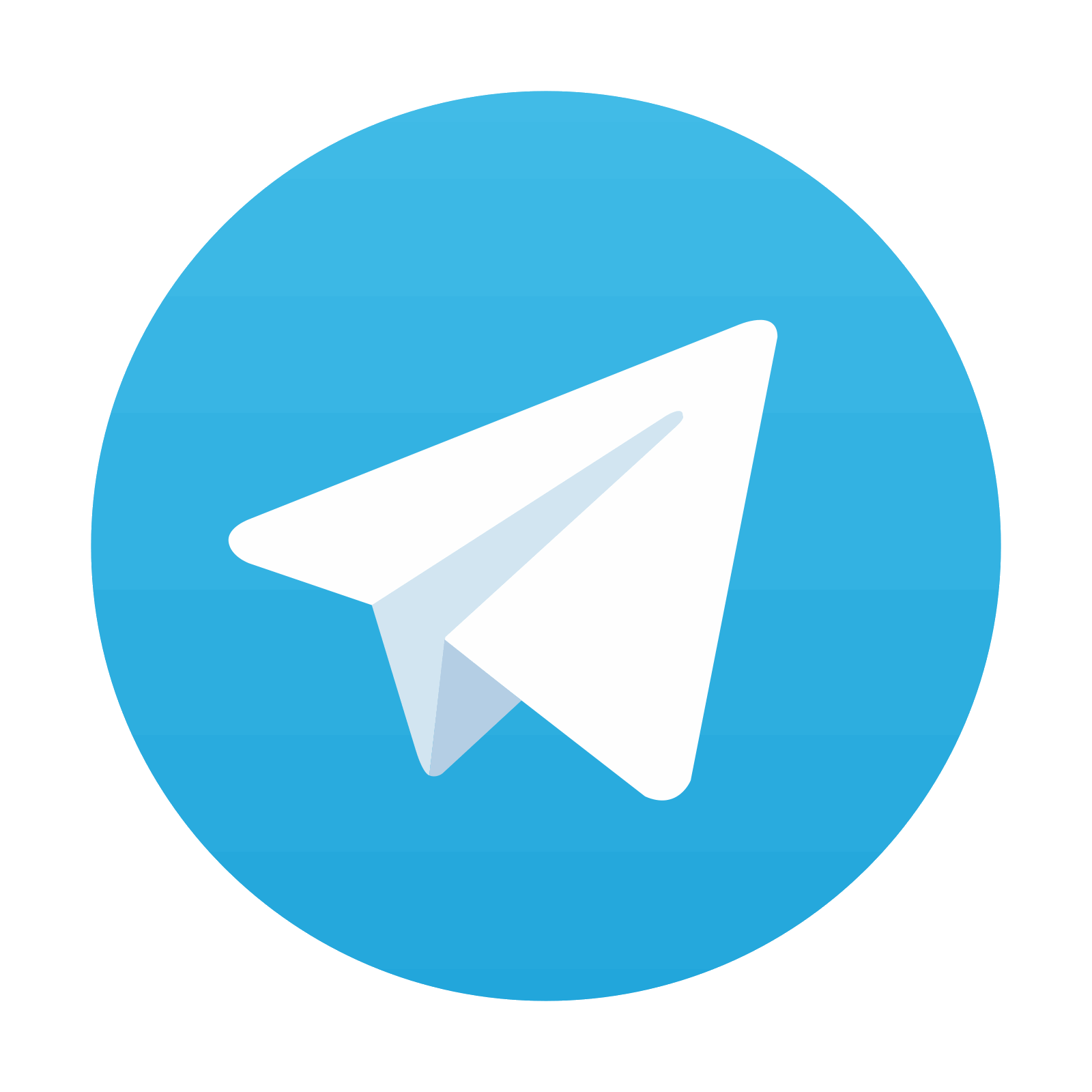
Stay updated, free dental videos. Join our Telegram channel

VIDEdental - Online dental courses
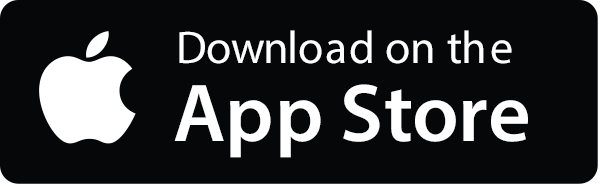
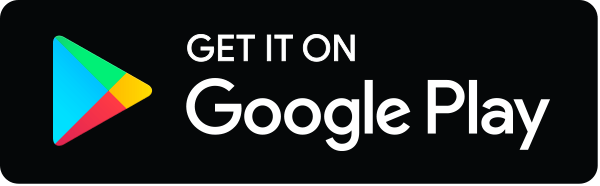
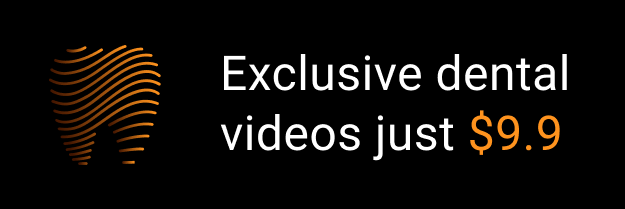