Abstract
Objectives
The aim of this study was to investigate the effects of polymerization contraction, shrinkage stress and Young’s modulus of nanofiller containing resin composites on early marginal adaptation of restorations in cavities.
Methods
Six nanofiller containing and two reference resin composites were studied. Marginal gap widths of restorations in cylindrical 4.2 mm wide and 1.5 mm deep dentin cavities, non-bonded or bonded with a self-etch adhesive, and in Teflon cavities of same dimensions were determined 15 min after irradiation ( n = 8). Polymerization shrinkage strains were measured using the bonded-disk ( n = 8) and a strain gage method ( n = 8). For determination of contraction stress the composites ( n = 10) were bonded to and cured in Araldit molds using a photoelastic method. Flexural moduli of the restoratives were studied according to ISO specification 4049 ( n = 5). Statistical analysis was performed with one- and two-way ANOVA, Kruskal–Wallis ANOVA test and post hoc tests ( p < 0.05).
Results
Only two nanofiller composites (Kalore, GC, Japan) and Venus Diamond (Heraeus Kulzer, Germany) showed consistently gap-free margins in bonded dentin cavities. The mean gap widths in non-bonded and in Teflon cavities were 6.1–12.8 and 14.1–25.5 μm, and linearly correlated ( r 2 < 0.85). Significant linear relationships were observed between strain, stress and marginal gap widths in non-bonded and Teflon cavities ( p < 0.01). Flexural moduli (15 min) were between 1.66 and 8.63 GPa.
Significance
Marginal cavity adaptation of restorations in bonded dentin cavities reflects complex interactions between adhesive bonding on the one hand, and polymerization contraction strain, stress and elastic modulus, on the other.
1
Introduction
During recent years a steadily increasing number of resin composites containing nanofiller particles have been introduced to the dental marketplace. The term “nano” seems to be very fashionable and is extensively used by manufacturers without readily perceivable justification. These products are often characterized as low-shrinkage restorative resins, thus insinuating improved adaptation to bonded cavity walls and in consequence better long-term clinical performance. This claim is however not substantiated by the few published clinical reports comparing nanofiller containing composites with conventional hybrid-type composites . Both types of resin composites performed satisfactorily after a few years in service, although secondary caries was still the main reason for restoration failure. Therefore, polymerization shrinkage remains still a major challenge to the longevity of resin composite restorations.
As with conventional composites the monomers included in nanofiller containing resins are dimethacrylates. Hence, upon activation the intermolecular spaces between monomers are reduced by conversion of C C bonds to covalent C–C bonds. Such polymerization shrinkage leads to stress as soon as the gel-phase is reached, where the polymer gradually loses viscous flow and becomes an elastic solid. With increasing post-gel polymerization contraction the stiffness of the polymer and the contraction stress increase, at a high stress rate and rapid gain in rigidity during the early stage of polymerization . Adverse clinical consequences of the shrinkage stress are tooth/cavity deformation and cuspal movement , failure at the composite-cavity interface , post-operative sensitivity , microleakage , and in the long-term caries .
Several authors evaluated the interactions of polymerization contraction, stress and rigidity using a cavity model for determination of the gap dimensions along the cavity margin and/or the cavity wall and the resin-based restorative . Such a model is useful to demonstrate the overall effect of resin polymerization shrinkage; however the effects of individual parameters related to the curing process such as contraction, stress and rigidity cannot be separated.
Several methods have been suggested to investigate curing shrinkage kinetics of resin composites. Watts and Cash have described a “bonded-disk” method for registration of curing contraction, calculating volumetric contraction from the linear displacement of a thin glass disk placed on a brass ring with the resin composite sample located centrally. Alternatively, the linear curing shrinkage of resin composites is investigated with so-called linometers. Typically, the composite is placed between two opposing rods, attached to a load cell and the movable traverse of a testing machine . Other report describes measurements of linear dimensional changes of curing resin composites using light microscopy . Recent more sophisticated methods for evaluation of the amount of polymerization contraction are digital holographic interferometry , X-ray microcomputed tomography , a procedure that enables measurement of resin volume shrinkage on clinically relevant geometries, and digital image correlation . Strain gages proved to be simple yet sensitive measuring tools for determination of post-gel polymerization contraction of composites .
Polymerization contraction creates stresses in the restorative material, at the interface to the surrounding tooth, and in the tooth substrate. The amount of contraction stress created depends on various factors, i.e. the shrinkage magnitude, the flow of the resin composite, the elastic modulus of the setting restorative, the bonding to the surrounding cavity walls, the cavity size and geometry (C-factor), and the application and processing techniques . Shrinkage stress can be partly relieved by resin composite flow relaxation depending on composition of the restorative, in particular on type and amount of filler particles included . Both, kind and content of filler, and composition of the resin matrix are determinants of the amount of shrinkage and stress, and of the resulting polymer stiffness that rapidly increases when the paste is converted from viscous compound to elastic solid. Therefore, contraction stress kinetics have been evaluated intensively, using different evaluation methods, such as finite element analysis , photoelasticity , strain gages attached to the composite and different types of tensilometers .
With most of the testing methods mentioned the values obtained are relative figures that do not permit conclusions about the absolute magnitudes of strain and stress occurring in the cavity. In spite of this limitation these methods are suitable and convenient to investigate systematically parameters that influence on strain and/or stress of composites under different conditions.
Aim of this study was to investigate at an early stage after light activation effects of polymerization contraction, shrinkage stress and stiffness (Young’s modulus) of nanofiller containing resin composites on marginal adaptation of non-bonded and bonded composite restorations in cylindrical dentin cavities as well as in Teflon model cavities of same dimensions. The research hypothesis to be tested was that application of resin restoratives with low polymerization contraction, low contraction stress, and low stiffness would result in superior marginal cavity adaptation of composite restorations.
2
Materials and methods
The six nanofiller containing resin composites and two reference materials, a hybrid-type (FIL) and a microfilled composite (DUR), used in this study are listed in Table 1 , including their main compositions, as extracted from publicly available manufacturer information. For cavity bonding the recently introduced single-step self-etch adhesive iBond SE (ISE; Heraeus Kulzer, Hanau, Germany) was used according to manufacturer instructions and combined with all composites tested. Throughout the investigation the LED light-curing unit Translux PowerBlue (Heraeus Kulzer, Hanau, Germany) was used. The light intensity was regularly checked with a radiometer (Demetron/Kerr, Danbury, CT, USA) to secure an output of >650 mW/cm 2 .
Material | Shade | Type | Code | Manufacturer | Batch | Composition | ||
---|---|---|---|---|---|---|---|---|
Monomer | Filler | |||||||
Composition | Vol.% | |||||||
Filtek™ Supreme XT | A3B | Nanofilled | FIL | 3M ESPE, St. Paul, MN, USA | 9WT | Bis-GMA, UDMA, Bis-EMA, TEGDMA | Aggregated SiO 2 /SrO 2 clusters (0.8–1.4 μm) an non-agglomerated SiO 2 (20 nm) | 59.5 |
Grandio | A3 | Nano-hybrid | GRA | Voco, Cuxhaven, Germany | 0913086 | Bis-GMA, TEGDMA | Fluorosilicate glass, SiO 2 (microfiller < 1 μm, spherical nanofiller < 5 nm) | 71.4 |
Kalore | A3 | Nano-hybrid | KAL | GC Corporation, Tokyo, Japan | 0906021 | UDMA (DuPont), DMA, UDMA | Prepolymer (incl. 400 nm SrO 2 and 100 nm lanthanoid fluoride). F–Al–silicate (700 nm), Sr–Ba–glass (700 nm), SiO 2 (16 nm) | 69 |
MI Flow | A3 | Nano-hybrid | MIF | GC Corporation, Tokyo, Japan | 0904132 | UDMA, DMA | Sr-glass, lanthanoid fluoride, SiO 2 (average = 700 nm) | 40 |
Tetric EvoCeram ® | A3 | Nano-hybrid | TET | Ivoclar-Vivadent, Schaan, Liechtenstein | L58159 | DMA, prepolymer (copolymer) | Ba-glass, YbF 3 , mixed oxide, prepolymer Size of inorganic fillers: 40–3000 nm, mean 550 nm |
53–55 |
Venus ® Diamond | A3 | Nano-hybrid | VED | Heraeus Kulzer, Hanau, Germany | 100027 | TCD-DI-HEA, PE-crosslinker | Ba–Al–F–silicate glass < 20 μm SiO 2 (5 nm) |
64 |
Filtek™ Z250 | A3 | Hybrid | Z250 | 3M ESPE, St. Paul, MN, USA | 9UR | Bis-GMA, Bis-EMA, UDMA | SiO 2 , ZrO 2 , particle size 0.01–3.5 μm, average 0.6 μm | 60 |
Durafill ® VS | A3 | Microfilled | DUR | Heraeus Kulzer, Hanau, Germany | 010210 | Bis-GMA, UDMA, TEGDMA | SiO 2 (20–70 nm) Prepolymer < 20 μm. SiO 2 in prepolymer: 32 wt.% | 66 |
2.1
Marginal gap determination in dentin cavities
For this trial 128 molar teeth, immersed immediately after extraction in 1% Chloramine T solution and stored for a maximum of 3 months, were used. Half of the teeth were randomly allocated to restoration of cavities without bonding, the other half to adhesive-bonded dentin cavities. Flat proximal dentin surfaces were produced by wet grinding on SiC paper, grits #180 through 600, until a sufficiently large peripheral area in coronal dentin was exposed for preparation of cylindrical but-joint cavities, 4.2 mm in diameter and 1.5 mm in depth. The cavities were prepared with a medium-grained diamond bur (approximately 10,000 rpm) under copious water spray. In the non-bonded group eight cavities each were rinsed with deionized water, slightly air-dried and filled with each of the eight resin composites from preloaded tubes, covered with a transparent matrix strip, light-cured for 40 s, and immediately immersed into deionized water at room temperature for 10–12 min. In the bonded group the adhesive ISE was applied to the rinsed and slightly air-dried cavities in one coat for 20 s agitated dwell time, thoroughly air-dried for 10 s, and light activated for 20 s. The eight cavities each were then bulk filled with the respective composite as described above.
Following removal of resin composite excess on SiC paper, grits 600–4000, the restoration margins were inspected under a light microscope at 500-fold magnification 15 min after start of light activation. When a gap was detected the width was measured, using an ocular screw micrometer with a reading accuracy of 0.2 μm. For each resin composite eight specimens were prepared.
Data sets were analyzed by one-way ANOVA and Tukey’s multiple comparison when normally distributed, or by non-parametric Kruskal–Wallis ANOVA and Mann–Whitney’s post hoc test for pairwise comparisons ( p < 0.05).
2.2
Marginal gap determination in Teflon cavities
Following a study design, presented by Irie et al. , 64 cylindrical cavities, 4.2 mm in diameter and 1.5 mm deep, were centrally lathe-cut in 15 mm wide and 10 mm high Teflon cylinders. Since Teflon does not interact with resin composite material this test procedure served to analyze the curing strain of the inserted resin composites. Eight cavities each were filled with each of the eight resin composites following the procedure outlined above for dentin cavities. Maximum gap widths, i.e. the sum of opposing gaps, were determined microscopically 15 min after start of light activation. The data were analyzed by one-way ANOVA and Tukey’s multiple comparisons at the 95% level of significance.
2.3
Polymerization shrinkage strain by “bonded-disk” method
Shrinkage was measured after placing 0.1 g (±0.005 g) of composite material on a microscope glass slide (76 mm × 26 mm × 3 mm). A stainless steel ring was placed around the specimen (inner 15 mm, width 3 mm, height 1 mm, tolerances ±0.02 mm) and a glass cover-slip (18 mm × 18 mm × 0.1 mm) was pressed gently into contact with the top of the ring using a parallel hand press. Depending on the density of the respective material the diameter of the resulting sandwiched specimen was 8.0 ± 0.2 mm. The assembly was then placed on a stainless steel specimen holder with a circular slot fitting the light-guide of the curing unit Translux PowerBlue (Heraeus Kulzer, Hanau, Germany) that was securely held in position by a metal clamp. The distance between the light-emitting window and the glass slide supporting the specimen was 0.5 mm. Once the assembly had been positioned under a chromatic scanner (MicroProf 200, FRT, Bergisch-Gladbach, Germany; measuring range for z -axis: 600 μm, accuracy 9 nm), the measuring spot of the transducer was aligned with the centre of the specimen. Subsequently, the resin specimen was light-cured from the below through the microscope slide for 20 s. Testing was done at ambient laboratory atmosphere.
CWL-software (FRT, Bergisch-Gladbach, Germany) was used to determine the linear change of path, i.e. the deflection of the cover-slip and specimen throughout 15 min after start of irradiation ( n = 6 per material). The following formula was used to calculate the percentage change in length of the composite specimen: Δ l = [( l x − l 0 )/ l 0 × 100] where l 0 is the original specimen thickness and l x the thickness of the resin specimen at time x after start of light activation.
Statistical data analysis was performed by one- and two-way ANOVA and Tukey’s post hoc test ( p < 0.05).
2.4
Polymerization shrinkage determined by strain gages
The strain gage method as described by Sakaguchi et al. was used to determine the post-gel shrinkage of the eight resin composites at ambient laboratory atmosphere.
Biaxial foil resistance self-temperature-compensating strain gages (KGF-3-120-D16-23L1M3S, 120 Ω gage resistance, gage factor 2.15, Kyowa Electronic Instruments, Tokyo, Japan) were placed on a microscopic glass slide. The grid length was 3.0 mm, grid width 1.3 mm, and the base diameter of the foil 10 mm. Approximately 0.1 g of composite resin was applied on top of the strain gage, resulting in a sample thickness of approximately 1.8 mm. The light-emitting window of the curing unit, clamped in a stand, was located centrally over the composite applied at a distance of 4 mm from the gage surface. The composite sample was then light-cured for 20 s with Translux PowerBlue. Dimensional changes of each composite ( n = 8) during and after irradiation (2, 5, 15 min, 1 and 24 h) were recorded by a personal computer through a sensor interface (PCD-300B, Kyowa Electronic Instruments) as changes in resistance and calculated as linear percentage shrinkage using the formula (Δ R / R )/ k × 100 = (Δ L / L × 100), where Δ L is the change in length, L is the original length, Δ R is the change in resistance, R is the original resistance and k is the gage factor.
Data were subjected to one- and two-way ANOVA and Tukey’s post hoc test at p < 0.05.
2.5
Determination of polymerization contraction stress
Shrinkage stress was determined by the method described by Ernst et al. . Cylindrical holes (4 mm in diameter) were prepared in Araldit B epoxy resin plates (40 mm × 40 mm × 4 mm), pretreated with the silicating system Rocatec Plus (3M ESPE AG, Seefeld, Germany) at a pressure of 2 bar, and conditioned with the silane coupling agent ESPE-Sil (3 M ESPE). Following 5 min drying time at ambient laboratory atmosphere the silane-treated surface was thinly coated with the light-curing bonding agent Visio Bond (3M ESPE) and irradiated for 20 s to ensure firm bonding of the resin composite to the wall of the mold.
The pretreated molds were placed on a glass plate on top of a transparent plastic strip, filled with resin composite from a preloaded tube, covered with another plastic strip and pressed flush under a parallel press. The specimens ( n = 10 per material) were then light activated from one surface with the light exit window of the curing unit Translux PowerBlue placed on top of the plastic strip for 60 s. The cured specimens were immediately immersed in deionized water (23° C) and transferred to a workstation on a stereomicroscope (Stemi 2000C, Carl Zeiss AG, Oberkochen, Germany) for photoelastic image records after 15 min, 1, and 24 h water storage. The digitally recorded pictures were analyzed on a computer to identify the localization and diameter of first order isochromatic curves, from which the polymerization shrinkage stresses (MPa) were calculated.
Data analysis was performed by one- and two-way ANOVA and Tukey’s post hoc test at p < 0.05.
2.6
Determination of flexural modulus
Five specimens of each resin composite were prepared for determination of flexural moduli after 15 min and 24 h storage in 37 °C water, respectively, following the procedure outlined in ISO specification 4049 . The specimens were prepared in molds (25 mm × 2 mm × 2 mm), placed on and covered with polyester foils, irradiated from both sides with five overlapping footprints of 20 s each with the Translux PowerBlue prior to immersion into the water bath. Immediately before testing, material flashes were removed and the specimen dimensions were determined with a micrometer screw (Type 293-561, Mitutoyo, Kawasaki, Japan, accuracy ±1 μm). The samples were loaded by three-point bending in a universal testing machine (Zwick 1454, Zwick/Roell, Ulm, Germany) at a crosshead speed of 1 mm/min until fracture occurred. The moduli (GPa) were calculated from the linear portion of the load/deflection curve using the equation: FM = l 3 F /4 wh 3 d , where FM is the flexural modulus, l is the length between the supports, F is the load applied, w is the width of the specimen, h is the thickness of the specimen and d is the deflection at load F .
Statistical data treatment was performed by one- and two-way ANOVA and Tukey’s post hoc test at p < 0.05.
2
Materials and methods
The six nanofiller containing resin composites and two reference materials, a hybrid-type (FIL) and a microfilled composite (DUR), used in this study are listed in Table 1 , including their main compositions, as extracted from publicly available manufacturer information. For cavity bonding the recently introduced single-step self-etch adhesive iBond SE (ISE; Heraeus Kulzer, Hanau, Germany) was used according to manufacturer instructions and combined with all composites tested. Throughout the investigation the LED light-curing unit Translux PowerBlue (Heraeus Kulzer, Hanau, Germany) was used. The light intensity was regularly checked with a radiometer (Demetron/Kerr, Danbury, CT, USA) to secure an output of >650 mW/cm 2 .
Material | Shade | Type | Code | Manufacturer | Batch | Composition | ||
---|---|---|---|---|---|---|---|---|
Monomer | Filler | |||||||
Composition | Vol.% | |||||||
Filtek™ Supreme XT | A3B | Nanofilled | FIL | 3M ESPE, St. Paul, MN, USA | 9WT | Bis-GMA, UDMA, Bis-EMA, TEGDMA | Aggregated SiO 2 /SrO 2 clusters (0.8–1.4 μm) an non-agglomerated SiO 2 (20 nm) | 59.5 |
Grandio | A3 | Nano-hybrid | GRA | Voco, Cuxhaven, Germany | 0913086 | Bis-GMA, TEGDMA | Fluorosilicate glass, SiO 2 (microfiller < 1 μm, spherical nanofiller < 5 nm) | 71.4 |
Kalore | A3 | Nano-hybrid | KAL | GC Corporation, Tokyo, Japan | 0906021 | UDMA (DuPont), DMA, UDMA | Prepolymer (incl. 400 nm SrO 2 and 100 nm lanthanoid fluoride). F–Al–silicate (700 nm), Sr–Ba–glass (700 nm), SiO 2 (16 nm) | 69 |
MI Flow | A3 | Nano-hybrid | MIF | GC Corporation, Tokyo, Japan | 0904132 | UDMA, DMA | Sr-glass, lanthanoid fluoride, SiO 2 (average = 700 nm) | 40 |
Tetric EvoCeram ® | A3 | Nano-hybrid | TET | Ivoclar-Vivadent, Schaan, Liechtenstein | L58159 | DMA, prepolymer (copolymer) | Ba-glass, YbF 3 , mixed oxide, prepolymer Size of inorganic fillers: 40–3000 nm, mean 550 nm |
53–55 |
Venus ® Diamond | A3 | Nano-hybrid | VED | Heraeus Kulzer, Hanau, Germany | 100027 | TCD-DI-HEA, PE-crosslinker | Ba–Al–F–silicate glass < 20 μm SiO 2 (5 nm) |
64 |
Filtek™ Z250 | A3 | Hybrid | Z250 | 3M ESPE, St. Paul, MN, USA | 9UR | Bis-GMA, Bis-EMA, UDMA | SiO 2 , ZrO 2 , particle size 0.01–3.5 μm, average 0.6 μm | 60 |
Durafill ® VS | A3 | Microfilled | DUR | Heraeus Kulzer, Hanau, Germany | 010210 | Bis-GMA, UDMA, TEGDMA | SiO 2 (20–70 nm) Prepolymer < 20 μm. SiO 2 in prepolymer: 32 wt.% | 66 |
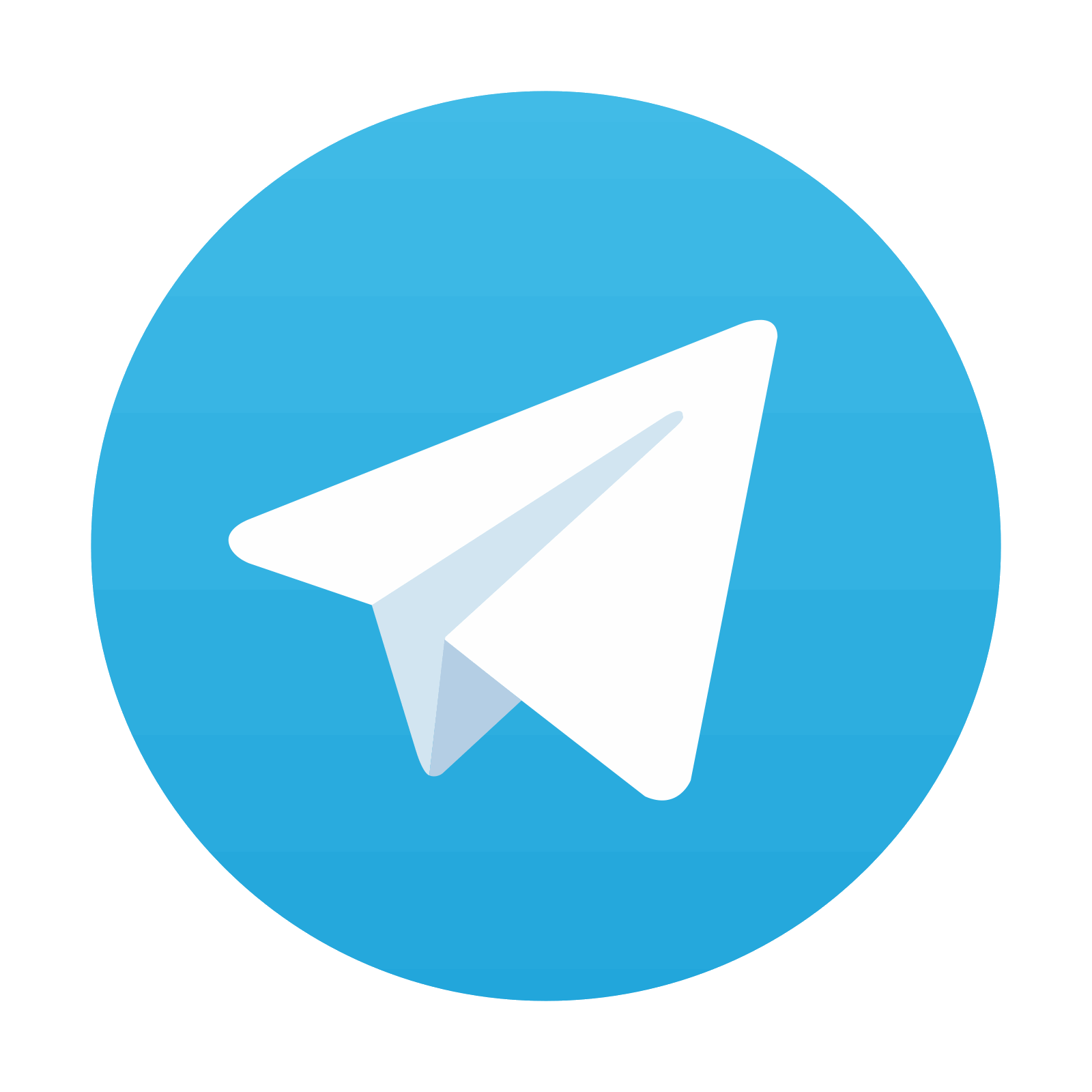
Stay updated, free dental videos. Join our Telegram channel

VIDEdental - Online dental courses
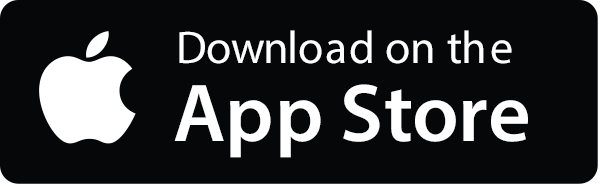
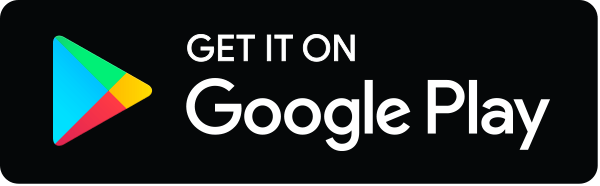
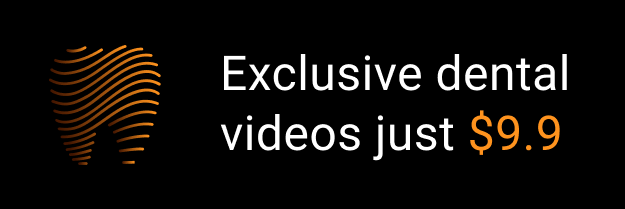