Abstract
Objectives
To detail the development of ester-free thiol–ene dental resins with enhanced mechanical performance, limited potential for water uptake/leachables/degradation and low polymerization shrinkage stress.
Methods
Thiol-terminated oligomers were prepared via a thiol–Michael reaction and a bulky tetra-allyl monomer containing urethane linkages was synthesized. The experimental oligomers and/or monomers were photopolymerized using visible light activation. Several thiol–ene formulations were investigated and their performance ranked by comparisons of the thermo-mechanical properties, polymerization shrinkage stress, water sorption/solubility, and reactivity with respect to a control comprising a conventional BisGMA/TEGDMA dental resin.
Results
The ester-free thiol–ene formulations had significantly lower viscosities, water sorption and solubility than the BisGMA/TEGDMA control. Depending on the resin, the limiting functional conversions were equivalent to or greater than that of BisGMA/TEGDMA. At comparable conversions, lower shrinkage stress values were achieved by the thiol–ene systems. The polymerization shrinkage stress was dramatically reduced when the tetra-allyl monomer was used as the ene in ester-free thiol–ene mixtures. Although exhibiting lower Young’s modulus, flexural strength, and glass transition temperatures, the toughness values associated with thiol–ene resins were greater than that of the BisGMA/TEGDMA control. In addition, the thiol–ene polymerization resulted in highly uniform polymer networks as indicated by the narrow tan delta peak widths.
Significance
Employing the developed thiol–ene resins in dental composites will reduce shrinkage stress and moisture absorption and form tougher materials. Furthermore, their low viscosities are expected to enable higher loadings of functionalized micro/nano-scale filler particles relevant for practical dental systems.
1
Introduction
Thiol–ene reactions have many attributes identified as a part of the “click” reaction paradigm . These include, among others, insensitivity to oxygen inhibition, high conversions coupled with fast kinetics, and mild reaction conditions . These robust reactions are very attractive in many potential applications including surface modification, biomaterials , polymer functionalization , photolithography , and nano- and micro-particle synthesis .
In the past decade, thiol–ene photopolymerization has gained significant attention as a candidate for dental restorative materials . Still, despite their high efficiency and many desirable properties, methacrylate-based resins continue to dominate the dental restoratives market. A widely utilized dental resin consists of 2,2-bis[ p -(3-methacryloxy-2-hydroxypropoxy)phenyl]propane (BisGMA) and triethylene glycol dimethacrylate (TEGDMA), which is present as a reactive diluent. BisGMA is an aromatic monomer with a rigid core structure that provides low monomer volatility and high polymer modulus. The high viscosity of BisGMA conveys several positive attributes but limits the amount of filler loading as well as the ultimate conversion. As a result, TEGDMA is incorporated as a reactive diluent, which at higher concentrations negatively affects shrinkage and the mechanical performance. Another disadvantage of using these monomers is the significant water sorption, and in consequence the potential for hydrolytic or enzymatic degradation, in particular of the esters. Degradation of monomers in conventional dimethacrylate-based dental restorative materials has been indicated in several adverse phenomena that result in reduced service lifetimes . Free-radical photopolymerization of dimethacrylates provides very rapid, on-demand curing under mild conditions. However, this polymerization exhibits a chain-growth propagation mechanism and is accompanied by substantial volume shrinkage and the associated shrinkage stress development that may cause clinical failure due to disruption of the bonded interface or deformation of the surrounding tooth structure .
Several studies have investigated the feasibility of formulating novel restorative materials that utilize the thiol–ene photopolymerization reactions . Due to the step-growth evolution of the network, the thiol and ene monomers are quickly consumed while low molecular weight species dominate in the early stages of the polymerization. This mechanism causes a delayed gel point conversion and lower polymerization induced shrinkage and shrinkage stress as opposed to chain-growth homopolymerizations. These previous studies have used primarily commercially available multifunctional thiols, mainly pentaerythritol tetrakis(3-mercaptopropionate) (PETMP). The photopolymerization of PETMP and triallyl-1,3,5-triazine-2,4,6-(1 H ,3 H ,5 H )-trione (TTT) was shown to occur at a much higher rate than BisGMA/TEGDMA cured under the same conditions. Most importantly, the maximum shrinkage stress achieved with the thiol–ene system was only 14% of the maximum shrinkage stress of the dimethacrylate system . This result motivated studies on alternative dental resin compositions such as oligomeric thiol–ene or ternary thiol–ene–methacrylate systems .
Recently we demonstrated that by eliminating the ester moieties from thiol monomers, non-degradable thiol–ene polymeric materials are effectively created with improved thermal and mechanical behavior. These materials also demonstrated extraordinary stability in acidic and basic solutions . Based on another study, we found that introducing a vinyl sulfone group as an ene moiety significantly enhanced the mechanical properties of the material . This array of approaches to improving mechanical and degradation properties was the motivation for the development of ester-free thiol–ene dental resins.
In this paper, we present ester-free thiol–ene resins with enhanced properties that will meet the strict requirements for dental materials, such as high functional group conversions, good mechanical behavior, low shrinkage and stress, and also with the unique benefit of the complete elimination of hydrolytically or enzymatically reactive chemical moieties. Thiol-functionalized oligomers were formed via thiol–Michael addition reactions using the synthesized tetra(2-mercaptoethyl)silane (SiTSH) and divinyl sulfone (DVS) monomers. Then, the resulting oligomers were photopolymerized with equimolar ene groups of trimethylpropane diallyl ether (TTT). In the second approach, monomeric thiol–ene resin mixtures were formed by using SiTSH, TTT, and a synthesized bulky tetra-allyl monomer containing urethane linkages. Polymerization induced shrinkage stress, reaction kinetics, mechanical properties, water sorption and solubility were evaluated for both the oligomeric and monomeric thiol–ene systems. Their performance was compared with a conventional BisGMA/TEGDMA dental resin as a control.
2
Materials and methods
2.1
Materials
Triethylamine (TEA), TTT, trimethylolpropane diallyl ether (DEA), tetravinylsilane, propylamine, thioacetic acid, and hexamethylene diisocyanate (HMDI) were purchased from Sigma-Aldrich. Divinyl sulfone (DVS) was purchased from Oakwood Chemicals. Irgarcure 819 (bis-(2,4,6-trimethylbenzoyl)-phenylphosphine oxide-BPO) was obtained from BASF. BisGMA/TEGDMA Solution Lot: 795-07 was purchased from ESSTECH. All chemicals were used as received. SiTSH and 1,3,5-tris-(3-mercaptopropyl)-1,3,5-triazine-2,4,6-trione (TTTSH) were synthesized according to a previously reported procedure . The tetra-allyl monomer (TENE) was synthesized upon treatment of an alcohol with an isocyanate to form urethane linkages . The structures of the thiol and vinyl monomers are depicted in Fig. 1 .

2.2
Monomer synthesis
2.2.1
Synthesis of SiTSH
Thioacetic acid (28.8 g) was added slowly to a flask with tetravinyl silane (10.0 g). The flask was kept in an ice-bath. Azobisisobutylronitrile (AIBN) (0.776 g) was used as a radical-generating thermal initiator. The solution was stirred at 65 °C for 36 h. After this first synthesis step was completed, thioacetic acid was distilled under vacuum at 65 °C. Without performing any purification, methanol (50 mL) and hydrochloric acid (20 mL) were added to a flask with the crude product from the thiol–ene reaction step. The solution was stirred at 60 °C for 12 h. After the second step was completed, the solvent was distilled and the product washed twice with 5 wt.% sodium bicarbonate solution. Finally, the crude product was purified by column chromatography (silica gel, dichloromethane (DCM)/hexane 1:2) to give a colorless liquid.
2.2.2
Synthesis of TTTSH
To a flask with TTT (10.0 g), thioacetic acid (14.4 g) was added slowly in an ice-bath. After addition of AIBN (0.776 g), the solution was stirred at 65 °C for 36 h. After the reaction was completed, thioacetic acid was distilled under vacuum at 65 °C. No further purification was performed on the crude product. Methanol (50 mL) and hydrochloric acid (20 mL) were added to a flask with the crude product from the first step. The solution was stirred at 60 °C for 12 h. After hydrolysis was complete, the solvent was distilled and the crude product was washed twice with 5 wt.% sodium bicarbonate solution. Finally, the crude product was purified by column chromatography (silica gel, ethyl acetate/hexane gradient from 0:1 to 4:1) to give a colorless viscous liquid.
2.2.3
Synthesis of TENE
The monomer was prepared by reacting HMDI with DAE in a NCO/OH = 1/1 stoichiometric ratio. The addition was carried out in a 100 mL round-bottom flask equipped with a magnetic stirrer and nitrogen gas inlet at ambient temperature for 24 h. The isocyanate was added dropwise to DEA containing one/two drops of dibutyltin dilaurate as a catalyst. The reaction was considered quantitative when the NCO IR signal at 2250 cm −1 disappeared completely. Then, the monomer was dissolved in DCM and washed through a silica plug. After solvent removal under vacuum, the monomer was used without any further purification.
2.3
Oligomer preparation
The gel point conversion of step-growth polymerizations is determined using the following equation :
α = 1 r f A − 1 f B − 1
where f A and f B are the functionalities of the two monomers and r is the molar ratio of monomers present such that it is always less than or equal to 1.0. Monomers with a stoichiometric imbalance for which alpha is less that one will form a crosslinked polymer when reacted via a step-growth mechanism. Theoretically, to form non-gelled oligomers at full conversion, the gel point conversion (i.e., alpha) has to be greater than one. Hence, by reacting thiol and vinyl sulfone monomers with a sufficient excess of a thiol monomer will produce thiol-terminated oligomers with full incorporation of sulfone groups within the oligomer structure. More specifically, a 3:1 and 4:1 molar ratio of thiol-to-ene functional groups in the trithiol:divinyl sulfone and tetrathiol: divinyl sulfone mixtures, respectively, were used to form the oligomers. These oligomers were synthesized via thiol–Michael addition in the presence of a solvent (DCM). After forming a homogenous mixture of the comonomers, 1 wt.% TEA was added to initiate the thiol–Michael addition reaction, which proceeds exclusively via a step-growth mechanism. The resulting multifunctional thiol-terminated oligomers were used for further kinetic and mechanical evaluation in thiol–ene reactions with TTT.
2.4
Fourier transform infrared spectroscopy (FT-IR)
The real-time conversion during photopolymerization was measured via Fourier Transform Infrared Spectroscopy (Nicolet 6700 FT-IR) combined with a vertical light cable. The mixtures were cured in the FT-IR chamber using irradiation intensities of 30 mW cm −2 or 50 mW cm −2 (400–500 nm filter was used) at the surface of the sample. The visible light intensity was monitored by a radiometer (model IL1400A equipped with a GaAsP detector and a quartz diffuser). Near-IR was utilized to evaluate the functional group conversions during curing of 1 mm thick samples sandwiched between glass slides separated by appropriate spacers. More specifically, the first C C overtone signal at 6160 cm −1 was monitored during the FT-IR measurements.
2.5
Shrinkage stress measurement
Simultaneous conversion and polymerization shrinkage stress measurements were conducted using a tensometer (American Dental Association Health Foundation), which measures polymerization-induced stress using cantilever beam deflection theory. The sample is clamped between two silanized glass rods. The tensile force generated by the bonded shrinking sample causes the cantilever beam to deflect. The deflection of the beam is measured with a linear variable differential transformer (LVDT) and translated into the appropriate force based on the calibrated beam constant. To calculate the appropriate stress, the force corresponding to the beam deflection is divided by the cross-sectional sample area. Simultaneous conversion measurements are facilitated using near-IR (as described before) transmitted through the polymer sample via fiber optic cables. A more detailed description of the tensometer and conversion measurement can be found elsewhere .
2.6
Water sorption/solubility test
Water sorption tests were performed using disc-shaped specimens of 1 mm in thickness and 15 mm in diameter. The curing was performed in an IR chamber according to the methodology described above. The samples were dried in an oven at 37 °C to constant initial mass ( m i ). The thickness and diameter of each sample film were measured with calipers at 4–5 points, and the initial volume ( V i ) was calculated. The samples were then placed in distilled water at 37 °C. At regular time intervals (∼12 h), the samples were removed from the water and with paper towel the excess water was blotted away. After their mass was recorded, the samples were returned to water conditioning. The measurements were repeated until there was no significant change in mass. This final mass is referred to as the equilibrium saturation mass ( m s ). After determining the equilibrium saturation mass, the dimensions of each sample were measured again and the saturation volume was calculated ( V s ). Finally the samples were dried in an oven at 37 °C for 24-h cycles until the measured mass remained constant. After determining the desorption mass ( m d ), the thickness of each sample was measured with calipers at 4–5 points, and the desorption volume ( V d ) was calculated. The equilibrium solubility limit, s , and the equilibrium water sorption, w , were calculated according to the following equations :
s μ g m m 3 = m i − m d V i , w μ g m m 3 = m s − m i V i .
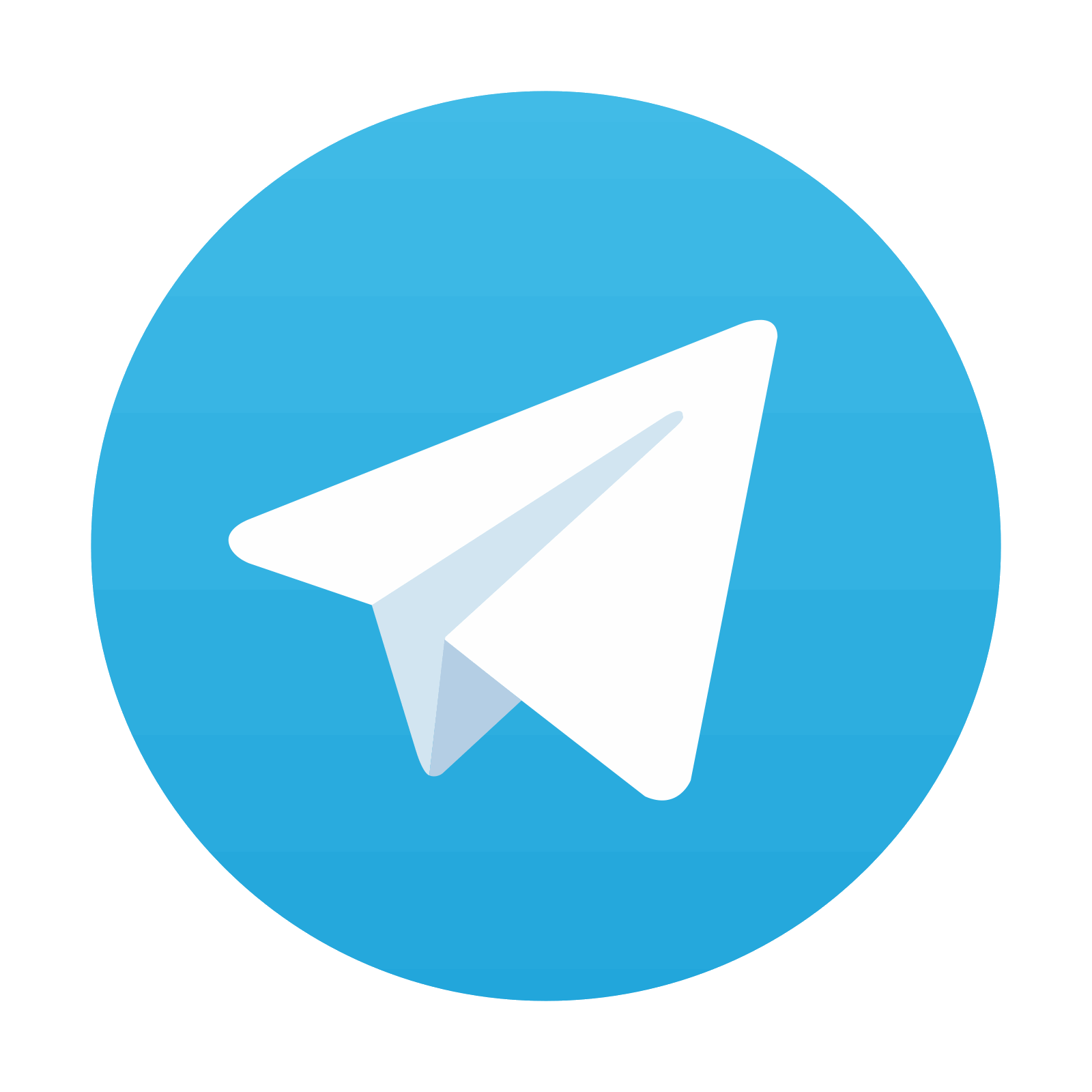
Stay updated, free dental videos. Join our Telegram channel

VIDEdental - Online dental courses
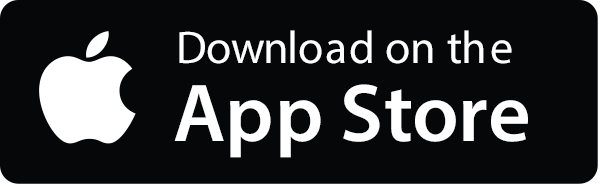
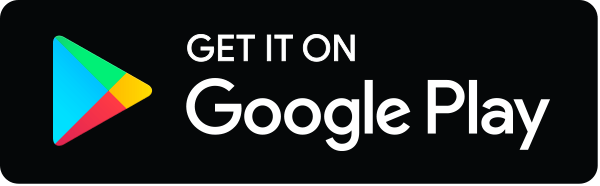