Abstract
Objective
This study aimed to investigate the effect of dentin surface moisture and curing mode on microtensile bond strength (MTBS) and nanoindentation characteristics of a self-adhesive resin cement.
Methods
Forty-four extracted human molars were distributed into four groups according to dentin surface moisture (dry or wet) and curing mode of the resin cement (light or chemical). Clearfil SA Cement (Kuraray Noritake Dental, Japan) was used for cementation of composite cores to the pulp chamber dentin. The specimens were sectioned into beams for MTBS test at the pulpal floor. Nanoindentation hardness and creep of the cement layer were measured under 100 mN load with 30 s hold segment. Data were statistically analyzed using two-way ANOVA and Weibull distribution of MTBS ( α = 0.05).
Results
Moisture, curing mode or their interaction did not significantly affect mean MTBS values that ranged 17.6–22.6 MPa ( p > 0.05); however, the lowest characteristic strength was found in moist chemically cured group. Hardness ranged 437–512 MPa, and was not affected by the experimental factors ( p > 0.05). Nanoindentation creep ranged 9.3–10.9% with the chemically cured groups showing the highest values, indicating lower cross-linking and deformation resistance of their polymer network.
Significance
Additional moisture on dentin surface did not contribute to adhesion of the anhydrous self-adhesive resin cement to dentin. Light-curing, despite attenuation through the composite core, was beneficial and improved nanoindentation creep resistance of the cement. The difference was not, however, reflected in the mean bond strength or hardness values.
1
Introduction
The success of an endodontically treated tooth relies not only on the apical sealing but is also highly dependent on coronal sealing. The adhesives used for bonding act as durable barriers hampering coronal microleakage, thus achieving an effective coronal seal, which is a fundamental step in prevention of bacterial invasion, secondary caries and decementation. It is also suggested that bonding techniques might have significant potential in enhancing the fracture strength of endodontically treated teeth and preventing vertical root fracture .
Indirect adhesive procedures represent a substantial portion of esthetic restorative procedures, especially for restorations where a large amount of the natural tooth substance is lacking. However, bonding to intracanal and pulp chamber dentin and coronal tissues should still be optimized . Variation in the structure of the pulp chamber makes it a challenger bonding substrate; since it is not prepared during the endodontic procedures, this area does not have a dominant smear layer. Therefore, the effectiveness of the adhesive systems depends mostly on collagen-rich predentin, enlarged tubules, and the small amount of intertubular dentin . The bond strength values reported for pulp chamber dentinal surfaces were usually lower than the bond strength values of flat coronal–occlusal dentin . Until recently, all luting agents required some pretreatment of the dentin; either acid-etching or application of a self-etching primer to prepare the tooth prior to cementation , which resulted in complex and technique sensitive application procedure. A new type of luting material has been developed that does not require any pretreatment of the tooth surface, the so-called self-adhesive resin cement (SARC) . Some of these materials rapidly gain popularity among clinicians due to their simplified application technique and favorable early results . In order to become widely accepted, the newly introduced SARCs are expected to offer good esthetics, optimal mechanical properties, dimensional stability, and good adhesion .
Dentin is composed of about 50 vol% mineral in the form of a carbonate rich apatite; 30 vol% organic matter which is largely type I collagen; and about 20 vol% water . Based on the intrinsically moist structure of dentin, bonding has been more complicated compared to enamel, especially with adhesive systems that require moisture control for optimal adhesion . On the other hand, while SARCs contain no water in their composition, water is crucial for their mechanism of action. The mechanism involves ionization of the acidic functional monomer to demineralize, penetrate, and establish a chemical bond with calcium ions form dentin apatite, simultaneously allowing for two-fold (i.e., micro-mechanical and chemical) bonding mechanism . SARCs have a limited ability to infiltrate dentin substrate due to their high viscosity compared to adhesive materials for direct bonding resulting in a relatively thin hybrid layer . Therefore, the role of chemical interactions such as those between the acidic functional monomers and the substrate , should be highlighted as adhesion mechanism for an SARC. Apart from the formation of complex compounds with calcium ions, different kinds of physical interactions, such as hydrogen bonding or dipole-to-dipole interactions were assumed to favorably influence self-adhesion .
The formulations of SARC are expected be moisture tolerant, as claimed by the manufacturers . SARCs rely on the intrinsic dentinal water and perhaps, water remaining on dentin surface after washing to establish adhesion. However, water content also affects the polymerization efficacy of resin blends depending on their chemical formulations and initiator mechanisms . Residual water may compromise integrity of the interface, where excessive moisture on the bonding substrate has been regarded as unfavorable . A previous report indicated that adhesion of an SARC was compromised to air-dried dentin surface and extra moisture resulted in better adhesion ; however, few other studies have investigated the effect of dentin moisture on the bond strength of other SARCs.
The SARCs are categorized as dual-cured resin cements, in which both light-activating and chemical-activating mechanisms are provided. Light activation of the resin cements may increase the degree of conversion when compared to chemical-activation alone and enhance physical properties . On the other hand, chemical-activation is expected to provide a uniform polymerization at the bottom of deep cavities where access for curing light is limited. Polymerization contraction of composite resin luting materials is known to produce high stresses in the interface, specially under constrained setup such as a in a cavity; with chemical activation alone, resin cements produced lower shrinkage and stress rates than the light-cured resins . The effectiveness of chemical activation without photo-irradiation of the resin cements has been variable among different resin cements, with some products exhibiting inadequate polymerization and poor adhesion when no light was applied . The acidic nature of an SARC has been mentioned as one factor hampering its effective polymerization specially under chemical mode .
Several techniques have been introduced to evaluate the mechanical performance of resins. More recently, nanoindentation technique has enabled investigation of local mechanical properties of materials under various loading regimes and allows the investigation of selected material properties on small amounts of materials, based on the load–displacement data of indentations on a submicron scale. Measurement of mechanical properties by nanoindentation has been suggested as advantageous over the conventional methods for its high resolution of force and accurate indent positioning . Hardness is known is as an indirect indication of its conversion degree . Resin-based dental adhesives exhibit time-dependent creep, which is likely to be related to the polymer network integrity and affect their performance . The time-dependent viscoelastic response was shown to be an important feature of both natural and synthetic biomaterials . Some previous studies have reported on the effect of curing strategy on mechanical properties of resin cements, but few studies have looked into indentation creep.
Thus, the aim of this laboratory study was to investigate the MTBS, nanoindentation mechanical properties of an SARC used with two curing modes of bonding to pulp chamber dentin under different surface moisture conditions. The null hypotheses proposed were that the moisture of dentin surface does not influence the mean MTBS to dentin and nanoindentation mechanical properties of the cement, and curing mode of the cement does not affect its properties.
2
Materials and methods
2.1
Materials used in this study
A SARC; Clearfil SA Cement (Kuraray Noritake Dental, Tokyo, Japan) was evaluated in this study. It was used for cementation of a composite core; Clearfil DC Core Automix (Kuraray Noritake Dental). Chemical composition and batch number of the cement and composite resin according to the manufacturer are shown in Table 1 .
Material (LOT) | Material composition | Procedure |
---|---|---|
Resin cement | Paste A: Bis-GMA,TEGDEMA, MDP, hydrophobic aromatic dimethacrylate, silanated barium glass filler, silanated colloidal silica, dl -camphorquinone, benzoyl peroxide | After mixing the equal amounts of paste A and B, apply the cement paste mix to the restoration and cavity prepared |
Clearfil SA | Initiator | |
Cement, Universal A2 (0141AA) | Paste B: Bis-GMA, hydrophobic aromatic dimethacrylate, hydrophobic aliphatic dimethacrylate, silanated barium glass filler, silanated colloidal silica, surface treated sodium fluoride, accelerators, pigments | |
Resin composite | Catalyst; Bis-GMA, TEGDMA, silanized glass filler, silica microfillers, photo/chemical initiator | Dual-cure composite for core build up; 60 s light cure |
Clearfil DC Core (01016A) | Universal; TEGDMA, methacrylate monomers, silanized glass fillers, silica microfillers, photo/chemical initiator |
2.2
Specimen preparation
Specimen preparation is schematically illustrated in Fig. 1 . Forty-four intact human molars were collected after the individuals’ informed consent was obtained under the guidelines of the Ethics Committee of Tokyo Medical and Dental University, and stored in distilled water and used within three month after extraction. The teeth were disinfected by storage in 0.02% thymol solution for 24 h prior to the experiment. The teeth were thoroughly scaled using curettes to remove calculus and remaining tissue tags. The coronal portion of teeth above the cervical third and roots up to 1–2 mm below the furcation was removed with a low-speed diamond saw (Isomet; Buehler, Lake Bluff, IL, USA) under water cooling and then the pulp chamber roof was removed and standard access cavities were prepared by cutting the pulp chamber roof and walls, without touching the pulpal floor. The coronal dentin was further reduced by 400-grit silicon carbide (SiC) paper (Sankyo, Saitama, Japan) to reach a depth of around 2 ± 0.2 mm for the exposed occlusal surface (from top to pulp chamber floor). The root canals were cleaned by hand instruments and sealed by a small amount of gutta-percha (DiaDent, Choongchong Buk DO, Korea). Composite cores were prepared using an indirect technique, by filling the composite into the prepared cavity, with a water soluble separating gel containing 1% Hydroxy Ethyl Cellulose (Wako Pure Chemical Industries, Osaka, Japan) applied on the cavity surfaces as a spacer. The composite cores were light-cured from the occlusal aspect by a conventional tungsten–halogen light-curing unit, XL3000 (600 mW/cm 2 , 3M-ESPE, St Paul, MN, USA) for 40 s. After the cores were removed from the teeth, they were further cured for 40 s from different directions. The adherent surfaces of the composite cores were then treated with 37% phosphoric acid for 10 s, rinsed, dried, and then silanized with a mixture of one drop of Clearfil SE Bond primer and one drop of Clearfil Porcelain Bond Activator (Kuraray Noritake Dental).
The specimens were divided into four groups according to curing mode of the resin and moisture of dentin surface as follows; group 1 (ML): moist light-activated, group 2 (DL): dry light-activated, group 3 (MC): moist chemical-activated and group 4 (DC): dry chemical-activated. For moist dentin surface, the cavity was thoroughly washed with water and then a moisture-absorbing paper was used to remove the water, leaving the surface visibly moist and shiny. In the dry groups, the dentin was treated as described above, but air-drying with a three-way air syringe at 3 kg/cm 2 pressure was applied for 10 s, leaving dentin surface dry with no visible moisture. SARC was then dispensed as instructed by the manufacturer, and applied to the pulp chamber cavity by a plastic instrument. The cores were then inserted into the cavity under finger pressure of the same operator. For the light-curing mode (ML and DL specimens), photo irradiation was applied by the halogen light-curing unit (600 mW/cm 2 ); excess cement was removed from margins after 3 s of light curing and then curing continued for another 40 s. For chemical-curing mode (MC and DC specimens), the restoration was left in darkness for an initial 3 min, then the excess was removed and the restoration left in dark for an additional 5 min, according to the manufacturer’s instructions. The specimens in all groups were then stored in water at 37 °C for 24 h.
2.3
MTBS test
Thirty-two teeth were used for MTBS testing. Each specimen was embedded in a fast curing acrylic resin (Unifast, GC, Tokyo, Japan) to support the resin–dentin interface during cutting and enhance handling of the specimen. Each specimen was then sectioned perpendicular to pulpal floor with the diamond saw to produce several slabs with approximate dimensions of 0.7 mm × 0.7 mm. Each slab was further sectioned to produce sticks, with bonding area of approximately 0.5 mm 2 . The central dentin sticks were used in order to eliminate substrate regional topological variability ( Fig. 1 (a, b)). The sticks acquired from the restored cavity were carefully inspected under a stereo microscope (Nikon SMZ1000; Nikon, Tokyo, Japan) to ensure proper orientation of the pulp chamber with regard to the force orientation for MTBS test. From each tooth, two central and suitable sticks were picked for the bond strength test ( n = 16).
In the selected beams, the exact cross-sectional area at the interface was measured with a digital vernier caliper (Series 406; Mitutoyo, Kawasaki, Japan) and then were attached to the testing jig of a handy-type universal testing machine (EZ-Test; Shimadzu, Kyoto, Japan) with a cyanoacrylate adhesive (Zapit; Dental Ventures of America, Anaheim, CA, USA) and subjected to MTBS testing at a crosshead speed of 1 mm/min. The value of load was divided over the cross-section area recorded as MTBS in unit of MPa.
2.4
Failure mode observation
To determine the mode of failure, all specimens were immediately examined after fracture under the Nikon SMZ1000 stereomicroscope at a magnification of 240×. The failure modes were classified into the following three types: adhesive failure at the resin cement and dentin interface (A); cohesive failure in resin cement or dentin; (C) mixed failure, a mixture of the described modes (M).
2.5
Nanoindentation
For mechanical properties evaluation of the cement at each group, twelve teeth were used. In the same manner as MTBS test, the specimens were divided into four groups of ML, DL, MC, and DC. Two central 2 mm-thick slices were obtained from each restored teeth by cross-sectioning along the central axis using the low-speed diamond saw under water cooling. The cross-sectional surfaces were then fine-polished by a polishing device (ML-160 A, Maruto, Tokyo, Japan) using 800, 1000, 1200, 1500, and 2000-grit SiC papers (Sankyo) and paper polishing pads under diamond paste slurries of particles 6, 3, 1 and 0.25 μm in diameter to create a very smooth surface suitable for the nanoindentation study. The specimens were inspected under confocal laser scanning microscope (CLSM; 1LM21H/W, Lasertec, Yokohama, Japan) prior to the test to check the integrity and the quality of the polished specimens. In this manner, 6 slices were prepared for each experimental group. The mechanical properties were obtained and compared among groups with a total of 30 indents over the entire length of the resin cement layer on the 6 slabs in each of the four groups, with a minimum increment of 50 μm between each two indents. Similar indentations were also performed on dentin below the cement layer to serve as a reference for nanoindentation creep displacement and hardness. A schematic description of this setup is presented in Fig. 1 .
Nanoindentation was carried out measured within the resin cement layer using a Berkovich diamond indenter attached to a nanoindentation device (ENT-1100a, Elionix, Tokyo, Japan). In the loading cycle, the maximum force of 100 mN was reached at 10 mN/s loading rate and held for 30 s prior to unloading ( Fig. 3 a).
Nanoindentation Martens hardness ( H M ) was calculated by dividing the maximum force by the projected area and then converted to MPa;
H M = P A P
where A P is the projected area under the maximum load ( P ). The calculations were performed according to the functions provided by the manufacturer of the nanoindentation device.
Nanoindentation creep ( C IT ) was defined as the relative change of indentation depth while the applied load remained constant during the holding time;
% C IT = h 2 − h 1 h 1 × 100
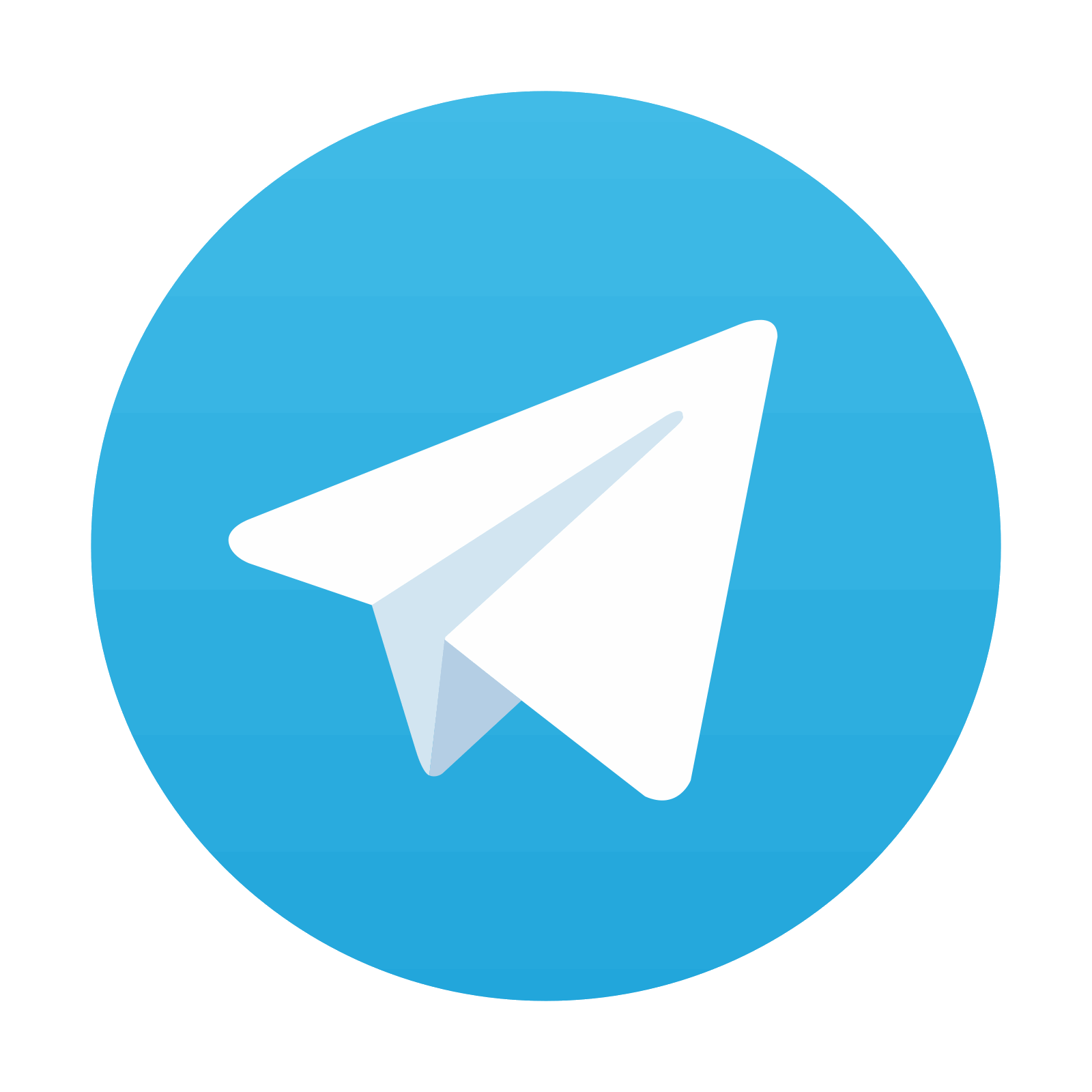
Stay updated, free dental videos. Join our Telegram channel

VIDEdental - Online dental courses
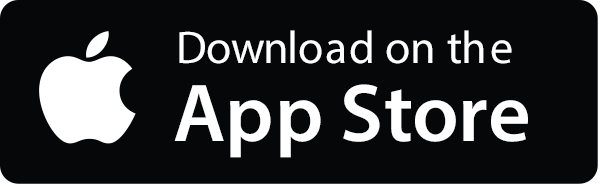
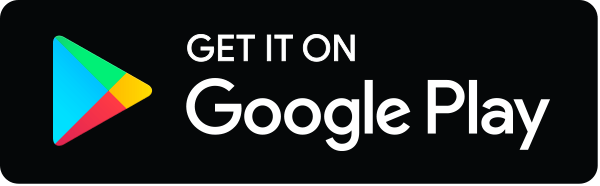