Highlights
- •
Each light curing unit has an individual irradiance distribution.
- •
The surface hardness map corresponds directly to the irradiance distribution.
- •
Longer exposure times lift the hardness pattern to higher values.
- •
Longer exposure times do not lead to more homogeneity of the hardness distribution.
Abstract
Objective
An inhomogeneous irradiance distribution from a light-curing unit (LCU) can locally cause inhomogeneous curing with locally inadequately cured and/or over-cured areas causing e.g. monomer elution or internal shrinkage stresses, and thus reduce the lifetime of dental resin based composite (RBC) restorations. The aim of the study is to determine both the irradiance distribution of two light curing units (LCUs) and its influence on the local mechanical properties of a RBC.
Methods
Specimens of Arabesk TOP OA2 were irradiated for 5, 20, and 80 s using a Bluephase ® 20i LCU in the Low mode (666 mW/cm 2 ), in the Turbo mode (2222 mW/cm 2 ) and a Celalux ® 2 (1264 mW/cm 2 ). The degree of conversion (DC) was determined with an ATR-FTIR. The Knoop micro-hardness (average of five specimens) was measured on the specimen surface after 24 h of dark and dry storage at room temperature.
Results
The irradiance distribution affected the hardness distribution across the surface of the specimens. The hardness distribution corresponded well to the inhomogeneous irradiance distributions of the LCU. The highest reaction rates occurred after approximately 2 s light exposure. A DC of 40% was reached after 3.6 or 5.7 s, depending on the LCU. The inhomogeneous hardness distribution was still evident after 80 s of light exposure.
Significance
The irradiance distribution from a LCU is reflected in the hardness distribution across the surface. Irradiance level of the LCU and light exposure time do not affect the pattern of the hardness distribution – only the hardness level. In areas of low irradiation this may result in inadequate resin polymerization, poor physical properties, and hence premature failure of the restorations as they are usually much smaller than the investigated specimens. It has to be stressed that inhomogeneous does not necessarily mean poor if in all areas of the restoration enough light intensity is introduced to achieve a high degree of cure.
1
Introduction
Visible light cured (VLC) resin based composites (RBCs) are commonly used as restorative materials . Adequate curing of these materials depends on the initiator receiving sufficient energy at correct wavelengths . Adjusting the optimum curing conditions by choosing correct light exposure time and irradiance level, precise positioning of the light curing unit (LCU) over the restoration site and not attempting to cure too much resin at one time make the difference between a resin that is properly cured and an inadequately cured restoration . The irradiance from the LCU has a significant influence on the surface hardness as well as on depth of cure (DoC) and degree of conversion (DC) .
The reactive radicals are created by the exposure of VLC RBCs. A photo-sensitive initiator, such as camphor quinone (CQ), absorbs light energy and changes into an excited state. The excited state is transferred to a reducing agent, usually an amine molecule e.g. dimethylaminobenzoate (DABE). The CQ–DABE-pair (exiplex) creates two free radicals. They attack the free carbon double bonds of the monomers and start the photo-polymerization. The number of generated radicals depends on the emitted irradiance of a LCU and affects thus directly the attainable DC .
The highest reaction rates and highest changes in DC were observed as long as the resin is below the glass-transition temperature ( T g ) . During the curing process T g increases because it is linked to the degree of polymerization of the resin molecules or the corresponding molecular weight, respectively. Therefore, T g exceeds the polymerization temperature after some time . The matrix transfers to the glassy state in which the mobility of the radicals and monomers is drastically reduced and the reaction rate tends to zero . Therefore, the existing radicals are trapped in the cured polymer matrix . After the exposure the trapped radicals either react very slowly with remaining carbon double bonds or deactivate over termination reactions over a long period of time. This effect known as “post-curing” leads to a further and slow increase of DC. It depends on the numbers of trapped radicals in the cured polymer matrix. Halverson et al. showed that the photo-polymerization process has an energy absorption limit. Over a specific energy level no improving of the mechanical behavior was observed .
Mechanical properties such as hardness, Young’s modulus or shrinkage depend directly on the DC . Several studies showed that a high DC corresponds to high hardness, high Young’s modulus, and high shrinkage . Therefore, possible irradiance distributions of LCU may produce inhomogeneous shrinkage resulting in internal stress distributions and/or debonding .
Several authors have described the problem of inhomogeneous irradiance output from LCUs, the effect on the curing of VLC RBCs and the consequences of the light output measurement as part of the quality management . Currently two different types of light-emitted diode (LED) LCUs are in use: Firstly, monowave blue-LED units emitting a relatively sharp blue light peak and secondly, polywave LED units emitting a broader spectrum with at least two peaks at different wavelengths. Some studies reported higher curing efficiencies in terms of hardness, elastic modulus or DC for polywave LCUs with the same or lower irradiance than for monowave LCUs .
Tungsten halogen lights and plasma arc lights also emit a broad range of wavelengths that cover the effective spectra of the commonly used photo-initiators . However, the classical tungsten halogen lights are being rapidly replaced by LED units due to convenience reasons and higher irradiances .
The surface hardness (Knoop or Vickers) has been used to characterize the mechanical properties of VLC RBCs . The DC within the resin was determined using Fourier transform infrared spectroscopy (FTIR) . Arikawa et al. and Price et al. found a correlation between the irradiance distribution and the hardness distribution .
However, the effects of prolonged exposure times on the hardness distribution requires further study as it is assumed that additional curing will occur in areas of lower DC as initiator radicals will have a slightly higher mobility there. This allows additional cross-links to be created in these areas leading to a more homogeneous polymer network. In most cases the exposure time will be between 5 and 40 s . The primary problems of current RBCs are inadequate polymerization, shrinkage and corresponding shrinkage stress as well as elution of low molecular substances. This restricts the durability of restorations and may induce allergies or other health implications .
Therefore, the hypotheses of this study were the follows:
- 1.
Each LCU has a specific irradiance distribution leading to a corresponding pattern of the hardness distribution of a dental composite surface.
- 2.
The number of radicals depends on the irradiance level and/or on the exposure time. A higher irradiance creates sufficient radicals to activate all carbon double bounds forming a more homogeneous network and longer exposure times provide radicals over a longer period. Thus, the effects of differences of irradiance distributions of LCUs are compensated by longer exposure time.
2
Materials and methods
2.1
Materials and sample preparation
A camphorquinone based VLC RBC – the micro-hybrid composite Arabesk TOP OA2, VOCO, LOT 1114471 (in the following text abbreviated Arabesk) – containing 22 wt.% bisphenol A glycidyl methacrylate (Bis-GMA), triethylene glycole dimethacrylate (TEGDMA), and urethane dimethacrylate (UDMA), and 77 wt.% of bi-ceramic system filler was used in this study .
2.2
Methods
2.2.1
Measurement of power and beam-profiles of the LCU
Two LED LCU with different emission spectra were used in this study, Fig. 1 :
- 1.
The monowave blue-LED LCU “Celalux ® 2” (Voco, Cuxhaven, Germany) delivering an irradiance of 1000–1500 mW/cm 2 , depending on the light guide tip (manufacturer information) and
- 2.
The polywave poly-LED LCU “Bluephase ® 20i” (Ivoclar Vivadent, Schaan, Liechtenstein) is used in Low mode with an irradiance of 650 mW/cm 2 and in Turbo mode with an irradiance of 2200 mW/cm 2 (manufacturer information) (in the following text abbreviated Low mode and Turbo mode) .

Both LCUs had a 10 mm entrance diameter and an 8 mm exit diameter turbo light guide tip.
The power output from each LCU was determined using an integrating sphere (LabSphere 6″) connected to an UV–vis spectrometer (Ocean Optics USB4000, Dunedin, FL). The power output P out was calculated by integrating the spectra between the wavelengths λ min = 350 nm and λ max = 550 nm, Fig. 1 .
The irradiance distributions across the tip of each light guide were determined using a Laser Beam Profiler (LBA USB-L070 Beam Profiler, Ophir-Spiricon, Logan, UT, USA) and evaluated with the Beam Gage software (Ophir-Spiricon). The LCUs were placed directly on a frosted glass shield (DG2X2-1500, Thor Laboratories, Newton, NJ, USA) in front of the camera lens, Fig. 2 . The intensity image of the beam-profiler in arbitrary intensity units has to be transferred to intensities with the calibration factor f cal :
f c a l = P o u t I t o t a l ⋅ A p i x e l
with the intensity value I total of the measured beam-profiler data and the area of a pixel A pixel with a linear dimension of 29.2 μm. Eq. (1) expresses f cal as irradiance per count.

Then the irradiance per pixel Ir pixel is given by
I r p i x e l = f c a l ⋅ I p i x e l
with I pixel as intensity per pixel in arbitrary intensity units.
The radiance exposure H e provides the information in which time the same amount of energy was delivered to the specimen surface for different LCU modes. It is defined by Eq. (3) :
H e = I r ⋅ t e
with the irradiance I r and exposure time t e .
2.2.2
Determination of the top hardness distribution
Micro-hardness specimens were made in 1 mm thick circular aluminum rings ( D out = 20 mm, D in = 8 mm). The aluminum rings were marked by a flat edge to ensure a defined and comparable position for each sample with respect to the light beam and so that they could be repeatedly placed in the same orientation in the Knoop micro-hardness tester. The Arabesk was carefully placed incrementally with a dental plugger into the center hole of the ring and covered with a Mylar ® strip to reduce air inhibition of the resin. A 1 mm thick microscope slide was used to press and flatten the sample to the thickness of 1 mm. The light guide tips were placed directly on the microscope slide over the center of the sample. The specimens were irradiated for 5, 20 and 80 s through the microscope slide and the Mylar strip using each LCU. For light exposure times exceeding the available time program, the LCU had to be restarted. Five specimens ( n = 5) were made at each light exposure time and with each LCU. The microscope slide and the Mylar strips were removed from the specimens 10 min after exposure.
Each specimen for the hardness tests was measured 24 h after exposure. They were stored in a dry and dark room at a temperature of (23 °C) for 24 h before Knoop micro-hardness was measured.
The micro-hardness (in the following text abbreviated hardness) distribution on the top surface of the specimens was measured with a Knoop micro-hardness tester (Mitutoyo, HM 101) equipped with an automatic xy -stage. The mapping was performed with 45 indentations with a lateral resolution of 1 mm on 5 specimens ( n = 5) according to Fig. 3 in order to calculate mean hardness and standard deviation for each coordinate point. The lateral resolution of 1 mm was chosen to affirm a threefold distance of the longest edges of the Knoop indentations being around 300 μm. This affirms that the indentations do not affect each other. The indentation force of 490.3 mN was applied for 8 s generating maximum indentation depths of 7 μm. The hardness was measured on the top surface because the intensity distribution of the LCU is most pronounced at the surface. Light scattering at the filler resin interfaces will partly change the lateral intensity distribution within the sample and complicate a correlation.

The results of the hardness mapping were compared with a two-sample test of variance (significant level α = 0.05) and the mean hardness was calculated as the arithmetic average for each indentation position. For each exposure time the mean of the hardness maps was compared with a two-sample t -test ( α = 0.05) to verify a significant difference between the different exposure times.
2.2.3
Determination of the DC by FTIR Spectroscopy
DC specimens were prepared in 1 mm thick Delrin ® rings ( D out = 15 mm, D in = 4 mm). The Delrin ® rings for the DC specimens were placed centrically over the Golden Gate ATR diamond (Bruker Tensor 27, Mid IR, FTIR). The Arabesk was carefully incrementally placed with a dental plugger into the center hole of the Delrin ® rings and covered with a Mylar ® strip to reduce air inhibition of the resin. A 1 mm thick microscope slide was used to press and flatten the sample to the thickness of 1 mm. The light guide tips were placed directly on the microscope slide over the center of the sample. The specimens were exposed with the Low and Turbo mode for 5, 20 and 80 s through the microscope slide and the Mylar strip using each LCU. For light exposure times exceeding the available time program, the LCU had to be restarted. The Mylar strip typically absorbed 5% of the LCU irradiation. Five specimens ( n = 5) were made at each light exposure time and with each LCU. Due to the experimental setup the DC had to be recorded at the bottom surface of the specimen on the ATR in real time for 180 s.
The curing process was investigated using a FTIR spectrometer (Bruker Tensor 27) equipped with an ATR-Golden-Gate camber (attenuated total reflection) under the following conditions:
Measuring time: | 180 s to determine the final DC |
Sample scan time: | 6 recording spectra in double side mode |
Resolution with respect to the wave number: | 8 cm −1 |
Scan rate: | 10 scans/s. |
A measure for the time dependent DC is in principle the ratio of the change of the absorbance band of the aliphatic C C double bound ( Abs ( Aliphatic ) ) with respect to the absorbance of the uncured state in the wave number range 1645–1620 cm −1 . Due to scatter between two measurements the absorbance of the aliphatic band is mostly normalized to the absorbance of the aromatic band ( Abs ( Aromatic ) ) in the wave number range 1620–1590 cm −1 because this band is not affected by the curing process.
DC determination is affected by the relationship between the molar ratio ( M r ) of Bis-GMA, TEGDMA, UDMA and their corresponding absorption ratio ( A r ).
A r = A b s ( A l i p h a t i c ) A b s ( A r o m a t i c )
The FT-IR equipment was calibrated based on a technique reported by Rueggeberg et al. using hydrogenated BIS-GMA and TEGDMA with different M r . The regression analysis of M r against A r showed a second order polynomial.
M r = 0.141 ⋅ A r 2 + 1.142 ⋅ A r
Then the DC is given by
D C = 100 % × 1 − 0.141 [ ( A b s ( A l i p h a t i c ) ) / ( A b s ( A r o m a t i c ) ) ] P o l y m e r 2 + 1.1424 [ ( A b s ( A l i p h a t i c ) ) / ( A b s ( A r o m a t i c ) ) ] P o l y m e r 0.141 [ ( A b s ( A l i p h a t i c ) ) / ( A b s ( A r o m a t i c ) ) ] M o n o m e r 2 + 1.1424 [ ( A b s ( A l i p h a t i c ) ) / ( A b s ( A r o m a t i c ) ) ] M o n o m e r
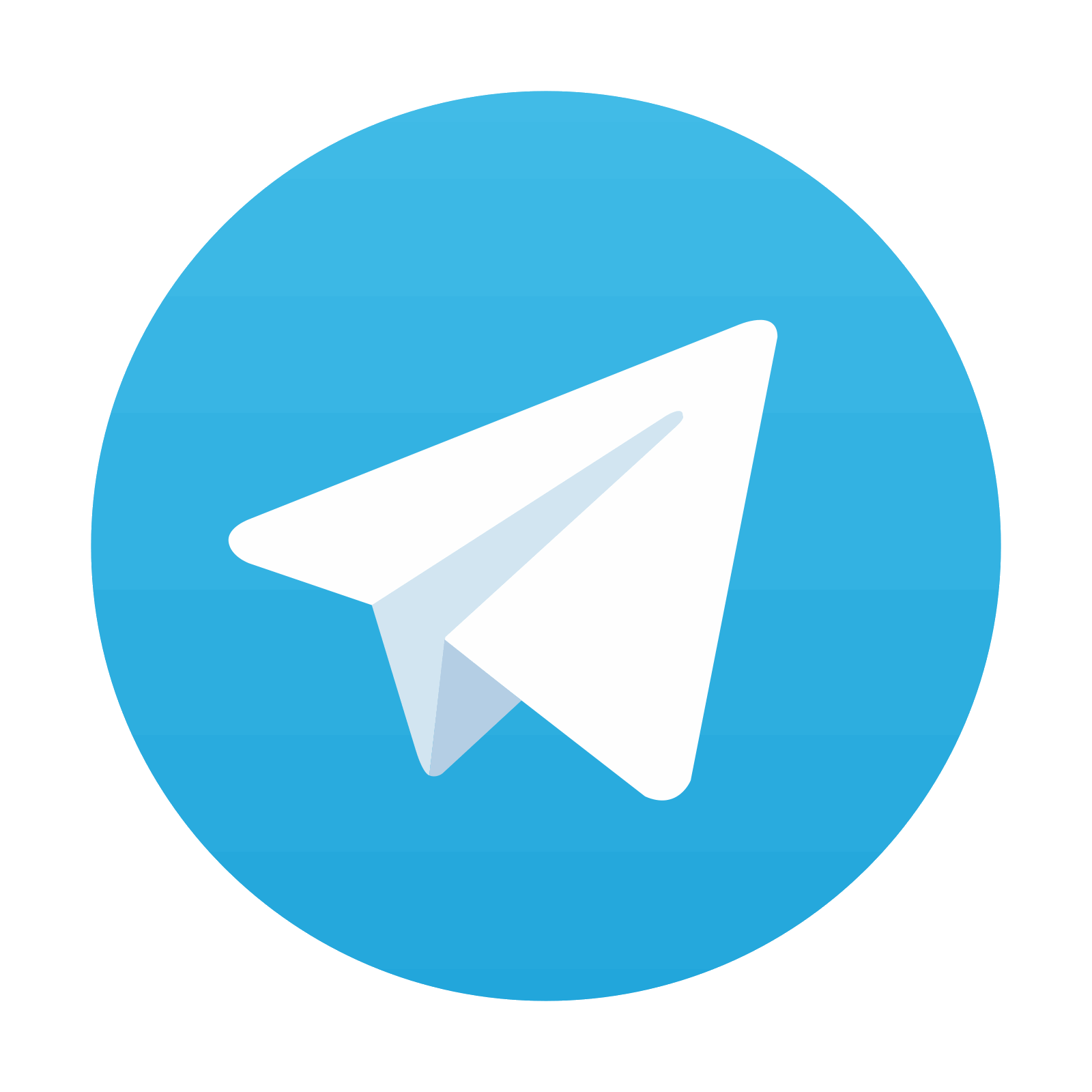
Stay updated, free dental videos. Join our Telegram channel

VIDEdental - Online dental courses
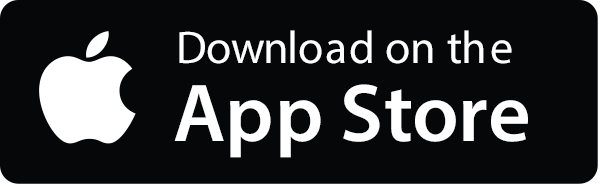
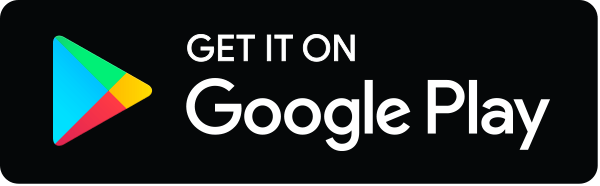