Abstract
Objectives
The biomodification of dentin is a biomimetic approach, mediated by bioactive agents, to enhance and reinforce the dentin by locally altering the biochemistry and biomechanical properties. This review provides an overview of key dentin matrix components, targeting effects of biomodification strategies, the chemistry of renewable natural sources, and current research on their potential clinical applications.
Methods
The PubMed database and collected literature were used as a resource for peer-reviewed articles to highlight the topics of dentin hierarchical structure, biomodification agents, and laboratorial investigations of their clinical applications. In addition, new data is presented on laboratorial methods for the standardization of proanthocyanidin-rich preparations as a renewable source of plant-derived biomodification agents.
Results
Biomodification agents can be categorized as physical methods and chemical agents. Synthetic and naturally occurring chemical strategies present distinctive mechanism of interaction with the tissue. Initially thought to be driven only by inter- or intra-molecular collagen induced non-enzymatic cross-linking, multiple interactions with other dentin components are fundamental for the long-term biomechanics and biostability of the tissue. Oligomeric proanthocyanidins show promising bioactivity, and their chemical complexity requires systematic evaluation of the active compounds to produce a fully standardized intervention material from renewable resource, prior to their detailed clinical evaluation.
Significance
Understanding the hierarchical structure of dentin and the targeting effect of the bioactive compounds will establish their use in both dentin-biomaterials interface and caries management.
1
Overview
Dentin is a complex mineralized tissue arranged in an elaborate 3-dimensional framework composed of tubules extending from the pulp to the dentin–enamel junction, intra-tubular, and peri-tubular dentin. The mineral portion is composed of carbonate apatites. Fibrillar type I collagen accounts for 90% of the organic matrix, while the remaining 10% consists of non-collagenous proteins, such as phosphoproteins and proteoglycans ( Fig. 1 ). The peri-tubular dentin, i.e., dentin surrounding the tubules, is highly mineralized (95 vol% of mineral), while most organic content is localized at the inter-tubular dentin (30 vol% of mineral) . Dentin undergoes modifications by physiological aging and disease processes to produce different forms of dentin . This process affects the biomechanics and biochemistry of the tissue.

Although similar in composition to bone, dentin does not share the same ability to remodel. This limits site regenerative therapies. An advantage of dentin over enamel is the presence of a collagen based scaffold that provides an appropriate cell-free backbone for tissue repair and regeneration. The presence of such a scaffold is a key to advance new concepts in tissue engineering approaches to the treatment of missing hard tissue. Recently, biomodification of dentin has been investigated as a biomimetic strategy therapy to mechanically strengthen the existing collagen network and also control biodegradation rates of extracellular matrix (ECM) components. This review provides an overview of important extracellular matrix components of dentin, as well as mechanisms and application of dentin biomodification, and specifically addresses the broad application of naturally occurring biomodification agents.
2
Extracellular matrix components relevant to dentin biomodification
2.1
Type I collagen
Fibrillar collagen is a strong and elastic biomaterial arranged into highly organized hierarchical structures . Type I collagen is the most abundant of all collagen types and is defined as a coiled-coil trimer molecule, each of which is composed of the repeated sequence of amino acids Gly-X-Y, where X and Y are commonly found to be proline and hydroxyproline, respectively. Type I collagen molecules are biosynthesized from a larger precursor, procollagen, by cleavage at both its C- and N-terminal ends. The collagen fibrils are formed by spontaneous self-assembly of the molecules into a periodic structure with 67–69 nm repeat period overlap between neighboring molecules, which is crucial for the development of covalent inter-molecular cross-linking. The inter-molecular cross-linking, the final post-translational modification of collagen, is the basis for the stability, tensile strength and viscoelasticity of the collagen fibrils ( Fig. 1 ). The slope of elastic stress-strain curve for collagen fibers increases with increased degree of cross-linking , and subtle perturbations to the cross-linking profile have been correlated with the strength of hard tissue . In addition, the biodegradability and thermal stability of the tissue is also controlled by the amount and type of collagen cross-linking.
Endogenous collagen cross-linkings are mediated by enzymatic and non-enzymatic reactions. Enzymatic intra- and inter-molecular cross-links, formed between telopeptides and adjacent triple helical chains through lysine–lysine covalent bonding , are controlled by a number of factors, such as lysine hydroxylation, glycosylation, turnover rate, molecular packing, and external forces . Non-enzymatic collagen cross-linkings are mediated by oxidation and glycation processes . Exogenous collagen cross-linking can be induced by non-enzymatic reaction sources such as chemical agents and physical methods, both of which have distinct mechanisms of interaction with type I collagen (see Section 3.1 ).
2.2
Proteoglycans
Proteoglycans (PGs) are a major group of non-collagenous proteins identified in both pre-dentin and dentin. PGs play a crucial role in dentin mineralization and the structural integrity of collagen fibrils . They are classified into two distinct categories: the large aggregating chondroitin/keratan sulfate family, composed of molecules such as versican and aggregan, and the family of small leucine-rich proteoglycans (SLRPs) . PGs found in dentin are mainly small leucine-rich collagen-binding carrying chondroitin-sulfate (CS, e.g. biglycan or decorin) with a limited distribution of keratan-sulfate (KS, e.g. fibromodulin, lumican) glycosaminoglycans chains (GAGs) . Although there are similarities in the structure of decorin and biglycan, they differ in the pre-dentin/dentin distribution and in related gene-expression during tooth mineralization . In addition to their roles in mineralization, PGs control the tissue hydration and molecule diffusivity . Hence, modified forms of the tissue, such as sclerotic dentin, can affect the distribution of PGs .
2.3
Endogenous proteases
Matrix metalloproteinases (MMPs) are a family of zinc-dependent endopeptidases; able to degrade different components of the ECM . In the oral cavity, MMPs are linked to periodontal disease, caries progression, pulp inflammation and cancer . In the dentin–pulp complex, several MMPs have been identified such as MMP-2, -3, -8 and -9 . Another important family of proteases are the cysteine cathepsins . Cathepsins belong to the papain family and were initially considered as lysosomal proteases, although they can act extracellularly . They become active at acidic pH and most are endopeptidases, with some exceptions like cathepsin B that can also act as a carboxypeptidase . Cathepsins participate in ECM degradation in physiological and pathological processes like bone remodeling, inflammation, rheumatoid arthritis, diabetes, multiple sclerosis and cancer . In addition to their role in caries progression , cathepsins have also been associated with other oral diseases such as periodontitis, bone resorption and oral cancer . Like MMPs, cathepsins are active in intact and carious dentin and show decreased activity with age in sound dentin .
2
Extracellular matrix components relevant to dentin biomodification
2.1
Type I collagen
Fibrillar collagen is a strong and elastic biomaterial arranged into highly organized hierarchical structures . Type I collagen is the most abundant of all collagen types and is defined as a coiled-coil trimer molecule, each of which is composed of the repeated sequence of amino acids Gly-X-Y, where X and Y are commonly found to be proline and hydroxyproline, respectively. Type I collagen molecules are biosynthesized from a larger precursor, procollagen, by cleavage at both its C- and N-terminal ends. The collagen fibrils are formed by spontaneous self-assembly of the molecules into a periodic structure with 67–69 nm repeat period overlap between neighboring molecules, which is crucial for the development of covalent inter-molecular cross-linking. The inter-molecular cross-linking, the final post-translational modification of collagen, is the basis for the stability, tensile strength and viscoelasticity of the collagen fibrils ( Fig. 1 ). The slope of elastic stress-strain curve for collagen fibers increases with increased degree of cross-linking , and subtle perturbations to the cross-linking profile have been correlated with the strength of hard tissue . In addition, the biodegradability and thermal stability of the tissue is also controlled by the amount and type of collagen cross-linking.
Endogenous collagen cross-linkings are mediated by enzymatic and non-enzymatic reactions. Enzymatic intra- and inter-molecular cross-links, formed between telopeptides and adjacent triple helical chains through lysine–lysine covalent bonding , are controlled by a number of factors, such as lysine hydroxylation, glycosylation, turnover rate, molecular packing, and external forces . Non-enzymatic collagen cross-linkings are mediated by oxidation and glycation processes . Exogenous collagen cross-linking can be induced by non-enzymatic reaction sources such as chemical agents and physical methods, both of which have distinct mechanisms of interaction with type I collagen (see Section 3.1 ).
2.2
Proteoglycans
Proteoglycans (PGs) are a major group of non-collagenous proteins identified in both pre-dentin and dentin. PGs play a crucial role in dentin mineralization and the structural integrity of collagen fibrils . They are classified into two distinct categories: the large aggregating chondroitin/keratan sulfate family, composed of molecules such as versican and aggregan, and the family of small leucine-rich proteoglycans (SLRPs) . PGs found in dentin are mainly small leucine-rich collagen-binding carrying chondroitin-sulfate (CS, e.g. biglycan or decorin) with a limited distribution of keratan-sulfate (KS, e.g. fibromodulin, lumican) glycosaminoglycans chains (GAGs) . Although there are similarities in the structure of decorin and biglycan, they differ in the pre-dentin/dentin distribution and in related gene-expression during tooth mineralization . In addition to their roles in mineralization, PGs control the tissue hydration and molecule diffusivity . Hence, modified forms of the tissue, such as sclerotic dentin, can affect the distribution of PGs .
2.3
Endogenous proteases
Matrix metalloproteinases (MMPs) are a family of zinc-dependent endopeptidases; able to degrade different components of the ECM . In the oral cavity, MMPs are linked to periodontal disease, caries progression, pulp inflammation and cancer . In the dentin–pulp complex, several MMPs have been identified such as MMP-2, -3, -8 and -9 . Another important family of proteases are the cysteine cathepsins . Cathepsins belong to the papain family and were initially considered as lysosomal proteases, although they can act extracellularly . They become active at acidic pH and most are endopeptidases, with some exceptions like cathepsin B that can also act as a carboxypeptidase . Cathepsins participate in ECM degradation in physiological and pathological processes like bone remodeling, inflammation, rheumatoid arthritis, diabetes, multiple sclerosis and cancer . In addition to their role in caries progression , cathepsins have also been associated with other oral diseases such as periodontitis, bone resorption and oral cancer . Like MMPs, cathepsins are active in intact and carious dentin and show decreased activity with age in sound dentin .
3
Biomodification of dentin – a bio-inspired strategy
Current therapies to replace missing tissue have primarily relied on synthetic biomaterials to restore function and shape of the tissue. Advances in tissue engineering and regeneration have grown exponentially in the past decades but with limited application in dental hard tissue repair resulting from the limited ability of the tissue to remodel. This site-specific limitation in tissue regeneration of dentin requires unconventional approaches to be used in an attempt to promote tissue repair. Therefore, any shift from a restorative to a reparative/regenerative dentistry will likely rely on better understanding of the tissue biochemistry, hierarchical structure ( Fig. 1 ), and implication to the biomechanics of the tooth.
One relatively small but significant step toward dentin tissue repair/regeneration is the development of a biomimetic strategy to enhance the tissue properties by modifying the chemistry of the tissue. The biomodification of existing hard tissue structures, specifically tooth dentin, is a novel approach to improve the biomechanical and biochemical properties of the tissue for preventive or reparative/restorative purposes. The approach was initially thought to be driven by non-enzymatic inter- or intra-molecular collagen cross-linking . However, the multiple interactions between bioactive agents with various extracellular components of the dentin matrix are likely the determinants of the tissue enhanced biomechanics and biostability. Therefore, the term biomodification is more appropriate to define the bioactivity of these highly bioactive chemical mediators.
3.1
Biomodification of dentin – sources and types
Type I collagen plays a major role on the replacement of missing tooth structure as the tooth component that provides micromechanical retention of resin-based materials and also as scaffold for tissue remineralization. Well-known synthetic agents, nature derived agents, and also physical methods have been shown to effectively interact with type I collagen and will be explored in this section. The sources and types of biomodification agents can be similarly classified as collagen cross-linking agents. Table 1 summarizes currently investigated agents for dental application. A brief overview of specific agents is given below with special emphasis on naturally occurring polyphenols due to their enhanced bioactivity, biocompatibility, and applications when compared to other well-known agents.
Types | Mediate non-enzymatic collagen cross-linking | Enzymatic inhibition | Proteoglycans | Dentin matrix interaction | |||
---|---|---|---|---|---|---|---|
Endogenous proteases | Exogenous proteases | Mechanical properties | Enzymatic degradation | ||||
Physical methods | |||||||
UVA Radiation/Riboflavin | + | + | N/N | N/N | N/N | + | |
Chemical agents | |||||||
Synthetic | Glutaraldehyde | + | + | + | – | +++ | + |
Carbodiimide/NHS | + | + | + | – | ++ | + | |
Naturally occurring | Genipin | + | – | N/N | N/N | + | + |
Polyphenols | |||||||
Oligomeric Proanthocyanidins | + | + | + | + | ++++ | + | |
Others Polyphenols | –/+ | + | + | N/N | –/+++ | + |
3.1.1
Synthetic resources
Physical methods : Also called the photo-oxidative method; most synthetic biomodifiers use light exposure, especially ultraviolet radiation . The photo-oxidative method requires the presence of singlet oxygen; and riboflavin (vitamin B2) is one of the most potent producers of oxygen radicals . Photo-activation of riboflavin results in singlet-oxygen-inducing chemical covalent bonds, bridging amine groups (N H) of glycine of one chain with carbonyl groups (C O) of hydroxyproline and proline in adjacent chains . Riboflavin is non-toxic and collagen cross-linking induced by UVA and radiation/riboflavin is a successful treatment for ophthalmic diseases such as keratoconus . While proven effective in the laboratory as a photo-activation method of riboflavin , safety issues regarding the use of UVA and its practicality for dental use need to be considered.
Chemical agents : Encompass the largest selection of potential synthetic mediators. Their established and postulated mechanisms of action are described below:
Aldehydes: While glutaraldehyde (GA) is the most widely known agent of this class, similar cross-linking ability is found with other aldehydes such as formaldehyde and glyceraldehyde. GA is a dialdehyde with high affinity for free primary amine groups of amino acids . GA has been associated with a decrease in the rate of collagen degradation and improved dentin collagen properties , reacting primarily with the ɛ-amino groups of peptidyl lysine and hydroxylysine residues of collagen fibrils. A disadvantage of GA is its high cytotoxicity , which limits clinical applicability.
Carbodiimide hydrochloride (EDC) is known as a zero-length agent due to its ability to cross-link peptides without introducing additional linkage groups. The cross-linking mechanism is mediated by the activation of carboxylic acid groups of glutamic and aspartic acids to form an O-acylisourea intermediate. The latter reacts with the ω-amino groups of lysine or hydroxylysine to form an amide cross-link, leaving urea as the terminal by-product. The addition of N -hydroxysuccinimide (NHS) to the EDC-containing solution is effective in increasing the number of induced collagen cross-linking and preventing the hydrolysis of activated carboxyl groups . While the cross-linking potential is limited , EDC is less toxic than GA .
3.1.2
Natural renewable resources
Also part of the family of chemical agents, naturally occurring compounds have received great attention in the past decade for their potential use in dentistry. Two of their most attractive characteristics are their very low toxicity when compared to synthetic agents and renewable/sustainable resources. Sources such as Genipin ( GE , an aglycone of an iridoid glycoside, one of the major components in the fruit of Gardenia jasminoides ) have found limited application due to its slow cross-linking reaction . Inter- and intra-molecular cross-linking is believed to be mediated by reactions with free amino acid (lysine, hydroxylysine, or arginine) to form a nitrogenous iridoid derivative that undergoes dehydration to form an aromatic monomer .
A large variety of bioactivities have been reported for polyphenols from plants. Their biological functions are the foundation for their prominent roles in plant-based dietary supplements and nutritionals, and recent research continues to explore new applications. Of particular interest to dental application are the proanthocyanidins (PACs) , belonging to a category known as condensed tannins, highly hydroxylated structures capable of forming an insoluble complex with carbohydrates and proteins . In addition to their dentin activity, PACs increase collagen synthesis, and are potent anti-microbial and anti-tumorigenics . The interaction of PACs and collagen results in the formation of complexes that are believed to be stabilized primarily by hydrogen bonding between the protein amide carbonyl and the phenolic hydroxyl in addition to covalent and hydrophobic bonds. The relatively high stability of PACs-protein complexes suggests structure specificity , which encourage hydrogen binding but also creates hydrophobic pockets . Such microenvironments decrease the dielectric constant enhancing the stability of such H-bonds. Non-specific inhibition of protease activity and interactions with proteoglycans have broadened the dental applications. Similar to other natural occurring agents, the source and preparation of PACs from the raw materials are essential for determining their interactions with the tissue. In an attempt to unravel this complexity, the section below provides comprehensive description of their chemistry, manipulation and characterization.
4
Proanthocyanidins
4.1
Polyphenol chemistry underlying dentin interactions
Polyphenols represent secondary metabolites which are produced by plants, likely as part of their defense mechanism. Different classes of polyphenolic compounds commonly observed in plants are phenolic acids, flavonoids (sub-classes: flavones, isoflavones, flavanols, flavanones, and anthocyanins), stilbenes, and lignans . An important aspect of the flavonoid chemistry is the formation of oligomers/polymers from monomers such as epicatechin or quercetin by mutual condensation, or conjugation with sugars to form O-glycosides (e.g., rutin = quercetin-3- O -rutinoside) or C-glycosides (e.g., epicatechin-8- C -β- d -galactopyranose in Theobroma cacao ) , and other phenolic acids like gallic acid by condensation reactions forming gallates e.g., epigallocatechin gallate in Camellia sinensis (green tea). These conjugated/condensed products can be easily confused with the monomers in the context of both their structure and nomenclature. For example, hesperidin, a flavanone glycoside, is derived from the aglycone hesperitin, with the only difference between the two compounds being the presence of a disaccharide, rutinose.
Proanthocyanidins (PACs) are divided into different classes based on the hydroxylation patterns in the A and B rings of the flavane skeleton. PACs containing (−) epicatechin (majority) or (+) catechin as their building blocks are known as procyanidins. The monomeric building blocks of proanthocyanidins are flavan-3-ol units composed of three rings ( Fig. 2 A ), which based on IUPAC rules are labeled on the basis of their biosynthesis (A – triketide, B – phenylpropanoid, C – pyran ring, formed by condensation). Oligomeric proanthocyanidins (OPACs) are most frequently composed of (−) epicatechin (2,3- cis ) and (+) catechin (2,3- trans ) terminal and extension units. The presence of chiral centers at positions 2 and 3 in the monomers and 2, 3, 4 in the dimers result in 4 and 8 stereoisomers, respectively. This imparts an exceptional amount of structural diversity and complexity to the already complicated biocombinatorial chemistry of the PACs ( Table 2 and Fig. 2 B).
In PAC oligomers and polymers, the monomers are linked together via A-type (C-4 → C-6/C-8 and C-2 → oxygen at C-7) and B-type (C-4 → C-6/C-8) linkages. The monomers range from 290 to 306 Da molecular weight (MW), while the OPACs range from 500 to 3000 Da. All PACs above 3000 Da are considered polymers whose MWs can reach up to 20,000 Da or even higher. As the molecular weight of PACs increase, they become increasingly polar due to the presence of additional hydroxyl groups and hence potential for hydrogen bonding. The polymeric PACs, which are frequently called “tannins”, have a tendency to form complex aggregates. They possess significant solubilization difficulties in commonly used polar organic solvents and are also known to precipitate proteins. These tannins have molecular weights in the range of 1000–5000 Da. There are two distinct and major classes of tannins: the hydrolysable tannins, containing hydrolysable ester bonds between the major building blocks, and the condensed tannins, in which the monomers are linked by C C or C O C bonds.
The hydrolysable tannins are classified into gallitannins and ellagitannins which are composed of gallic acid and ellagic acid units, respectively. These tannins can be hydrolyzed by acid or enzymes such as tannase. These polyphenols were primarily used by the leather industry for tanning purposes, but are also found in many plants of medicinal interest such as pomegranate rind and bark, bearberry leaves and cloves.
The condensed tannins are not readily hydrolysable like the gallitannins or ellagitannins, and glycosidation is not commonly observed . Most of the observed medicinal properties of tannins are attributed to the class of condensed tannins.
The third, relatively rarely studied class of tannins called the “complex tannins” consists of conjugations between PACs and hydrolysable tannins via C C bonds .
4.2
Structural diversity and chemical space of oligomeric plant phenols
4.2.1
Structures of oligomeric proanthocyanidins (OPACs)
OPACs are one of the two large groups of polyphenols, which consist of condensed polyphenolic moieties. Representing oligomeric or even polymeric condensates, the building blocks are flavan-3-ols which are mainly linked through C-4 → C-8 or C-4 → C-6 carbon-carbon bonds (B-type proanthocyanidins). Structures with an additional inter-flavane ether bond between C-2β → O → C-7 (or C-2β → O → C-5), they are classified as A-type OPACs . OPACs are divided into different subclasses depending on the specific structure of the monomeric flavan-3-ol units, which include catechin (C), epicatechin (EC), gallocatechin (GC), epigallocatechin (EGC), afzelechin (AZ), epiafzelechin (EAZ), and all their 3- O -gallic acid esters, i.e., catechingallate (CG), epicatechingallate (ECG), gallocatechingallate (GCG), epigallocatechingallate (EGCG), respectively . The most abundant types of OPACs are procyanidins containing catechin (C) and epicatechin (EC) subunits ( Fig. 3 ) .
From the perspective of 3D structural diversity, OPACs possess a large number of structural variations, which result from the different monomer types, the different inter-flavanoid linkages (bond types and linked positions), and degree of polymerization ( Table 3 ). The main flavan-3-ol units of grape seed PACs are C, EC, CG, and ECG, and these monomers are mainly linked with C-4 (α or β) → C-8 or C-4 (α or β) → C-6 inter-flavanoid bonds . Accordingly, there are 64 theoretical structural possibilities for grape seed PACs dimers, as calculated using the principle of permutation as shown in Fig. 2B. Table 3 provides an overview of the theoretical numbers of potential permutations in the OPACs of various plants under investigation in the authors’ laboratory. The calculations take into account the degree of polymerization (DP), the known types of monomers, and the linkages that have been described for each plant. While the assumption of a “combinatorial biosynthesis” approach may be an overestimate of the actual OPACs metabolomes, it still demonstrates the exponential increase in the number of theoretical structural variations of PACs oligomers, with thousands of variations already starting at the level of tetramers (DP = 4)( Fig. 3 ).
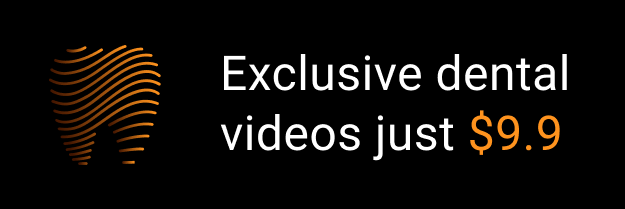