Abstract
Objectives
This study demonstrates the effects of nano-scale prepolymer particles as additives to model dental monomer and composite formulations.
Methods
Discrete nanogel particles were prepared by solution photopolymerization of isobornyl methacrylate and urethane dimethacrylate in the presence of a chain transfer agent, which also provided a means to attach reactive groups to the prepolymer. Nanogel was added to triethylene glycol dimethacrylate (TEGDMA) in increments between 5 and 40 wt% with resin viscosity, reaction kinetics, shrinkage, mechanical properties, stress and optical properties evaluated. Maximum loading of barium glass filler was determined as a function of nanogel content and composites with varied nanogel content but uniform filler loading were compared in terms of consistency, conversion, shrinkage and mechanical properties.
Results
High conversion, high molecular weight internally crosslinked and cyclized nanogel prepolymer was efficiently prepared and redispersed into TEGDMA with an exponential rise in viscosity accompanying nanogel content. Nanogel addition at any level produced no deleterious effects on reaction kinetics, conversion or mechanical properties, as long as reactive nanogels were used. A reduction in polymerization shrinkage and stress was achieved in proportion to nanogel content. Even at high nanogel concentrations, the maximum loading of glass filler was only marginally reduced relative to the control and high strength composite materials with low shrinkage were obtained.
Significance
The use of reactive nanogels offers a versatile platform from which resin and composite handling properties can be adjusted while the polymerization shrinkage and stress development that challenge the adhesive bonding of dental restoratives are controllably reduced.
1
Introduction
Extensive research work has been conducted regarding materials and light-curing methods designed to reduce the overall stress state in dental composites during constrained polymerization. This has included the development of a wide variety of novel monomers that provide reduced shrinkage compared with conventional dimethacrylates , use of compliant liners in dental restorations , and varied photo-activation protocols that alter reaction kinetics . Polymerization stress is related to polymer modulus and polymerization shrinkage while polymerization shrinkage is dependent on conversion and initial reactive group concentration . Therefore, a reduction in polymerization shrinkage while maintaining fixed modulus and conversion in the final polymer is expected to provide a proportional reduction in polymerization stress. In this investigation, high molecular weight polymeric nanoparticles (nanogels) are used as a swellable, potentially reactive additive in a secondary monomer with the goal to reduce the overall reactive group concentration and thereby reduce polymerization shrinkage and stress without compromise to other critical polymer properties.
Nanogels are internally crosslinked and cyclized single or multi-chain polymeric particles typically well below micrometer size. These materials have attracted substantial interest and have been prepared from very diverse monomer compositions by a number of synthetic routes designed to maintain discrete nanoparticle formation without macrogelation . Practical applications of nanogels include drug delivery, biosensing, microreactors, modifiers for coatings and polymer composites .
The use of prepolymerized particles in dental composites is obviously not novel since microfilled composites use an “organic filler” approach, whereby inorganic filler and resin are cured, crushed and added to monomer and filler to produce the final composite material. However, the particle size of the pulverized prepolymer is relatively large and the overall amount of filler that can be incorporated into these composites is limited, which results in higher coefficients of thermal expansion and water sorption as well as lower elastic moduli compared with conventional dental composites . In contrast, the use of nanogel prepolymer in composite applications may present a more versatile approach that allows a greater amount of inorganic filler to be incorporated due to the nanoscopic size of these prepolymer particles. Because the great majority of polymerization involving nanogels is performed prior to its introduction into a secondary monomer, the volumetric shrinkage in nanogel-modified materials is expected to scale inversely with nanogel loading. Nanogels bearing reactive sites, which may include both internal and surface-situated functional groups, have been prepared previously .
There are a number of examples of hyperbranched or dendritic macromers applied as components of dental restorative materials . The hyperbranched monomers used are generally well characterized structurally, but fairly low in molecular weight with a high degree of methacrylate group functionalization. Polymeric fibers produced by electrospinning processes represent another means to incorporate prepolymer into dental composites . There are no literature reports regarding the use of nanogel particles in dental polymers. The current study seeks to demonstrate a simple method to prepare reactive nanogels as well as the comprehensive evaluation of a model nanogel as an additive in triethylene glycol dimethacrylate (TEGDMA) as a model monomer matrix. The incorporation of inorganic filler in the nanogel-modified monomer is also examined to provide further relevance to dimethacrylate dental composite materials. This approach may offer a unique ability to controllably modify polymerization shrinkage, modulus and stress depending on the nanogel size, structure and fraction incorporated. The hypothesis tested is that substantial nanogel contents can be accommodated in both unfilled and filled resin formulations to reduce polymerization shrinkage and stress without detrimental impact to other critical material properties.
2
Materials and methods
2.1
Nanogel synthesis
Copolymer nanogels were synthesized by a batch process using a 70:30 molar ratio of isobornyl methacrylate (IBMA; Sigma–Aldrich Co., St. Louis, MO, USA) and urethane dimethacrylate (UDMA; Esstech, Essington, PA, USA), respectively. Free-radical polymerization was carried out in solution using a four-fold excess of toluene (relative to monomer mass) as a solvent. Bis(2,4,6-trimethylbenzoyl)-phenylphosphine oxide (BAPO, Irgacure 819; Ciba Specialty Chemicals, Basel, Switzerland) at 2.5 wt% was used as the photoinitiator. 2-Mercaptoethanol (ME; Sigma–Aldrich, 15 mol% relative to monomers) was included as a chain transfer agent to aid in the prevention of macrogelation as well as to provide sites for post-polymerization refunctionalization with methacrylate groups. Room temperature (23 °C) light-activation of the reactant mixture was conducted with an output irradiance of 2500 mW/cm 2 between 320 and 500 nm (Novacure; EXFO, Mississauga, Ontario, Canada) for approximately 2 h during which time, methacrylate conversion of greater than 90% was achieved (based on C C peak area at 1637 cm −1 relative to the C O absorbance at 1720 cm −1 ; includes both pendant vinyl groups and unreacted free monomer) as determined from the initial and final mid-infrared spectra (Nicolet 6700, Thermo Scientific, West Palm Beach, FL, USA). A foil-wrapped 100 mL round-bottom flask was used as the photoreactor with monomer batch sizes of approximately 10 g. Nanogel was precipitated from the clear reaction mixture by dropwise addition into an excess of hexane. The precipitate was filtered and the residual solvent was removed under reduced pressure to obtain the nanogel as a dry powder in approximately 85% yield. In order to obtain reactive, polymerizable nanogels, half of the isolated nanogel was added to a four-fold excess of methylene chloride to give a clear dispersion, which was mixed with a slight excess of 2-isocyanoethyl methacrylate (IEM; Sigma–Aldrich) (equimolar based on the original ME content) and stirred for 6 h in the presence of a trace amount of dibutyltin dilaurate (Sigma–Aldrich) as catalyst. The polymer precipitation method was repeated to isolate the methacrylate-functionalized reactive nanogel. The structures of the reagents used are shown in Fig. 1 .

2.2
Nanogel particle characterization
The polymeric nanogels were characterized by triple detector (differential refractive index, viscosity, light scattering) gel permeation chromatography (GPC; Viscotek, Houston, TX, USA) in tetrahydrofuran using a series of four columns spanning molecular weights of 10 4 –10 7 with absolute molecular weight based on right/low angle light scattering detection calibrated with a 65 kDa poly(methyl methacrylate) standard. As a control, IBMA was homopolymerized in solution with chain transfer agent under the same conditions used to prepare the nanogel. The glass transition temperature ( T g ) of the dry nanogel powder ( n = 2) was determined with a dynamic mechanical analyzer (DMA; Perkin Elmer 8000, Waltham, MA, USA) by sandwiching 10 mg of nanogel in a thin metallic pocket that was then subjected to single cantilever cyclic displacement of 50 μm at 1 Hz. The nanogel was heated to 180 °C with tan δ data collected as the sample was cooled to −50 °C at 2 °C/min in air. The density of the dry nanogel powder was determined using a helium-purged pycnometer (MVP-60C; Quantachrome Instruments, Boynton Beach, FL, USA).
2.3
Formulation of resins and composites
TEGDMA (Esstech) was used as a matrix monomer to be loaded with nanogel. The nanogel was loaded at 5, 10, 20, 30 or 40 wt%, for both reactive and non-reactive particles, with unfilled TEGDMA used as a control. Corresponding dual-filled resin composites were obtained by progressively loading the reactive nanogel-modified TEGDMA samples with the maximum capacity of barium borosilicate glass particles (0.4 μm average diameter) treated with 5 wt% of γ-methacryloxypropyltrimethoxysilane; Esstech) that could be incorporated homogeneously, based on visual inspection coupled with incremental filler addition (single determinations), using a centrifugal mixer (SpeedMixer DAC150; FlackTek, Landrum, SC, USA). In addition, the same TEGDMA/reactive nanogel series was loaded with a uniform barium glass filler content of 70 wt%. In order to render all these materials photo-curable with either UV or visible irradiation, 0.2 wt% of BAPO (relative to the resin content) was included in each mixture.
2.4
Viscosity and consistency
Viscosity measurements of the nanogel-modified TEGDMA materials were performed using a cone-plate digital viscometer (CAP2000+; Brookfield, Middleboro, MA, USA). A defined volume of the control and nanogel-modified monomer was tested at 25 °C under the following conditions: 200 rpm, 1 Hz, and a run time of 30 s. Consistency of the 70 wt% barium glass filled composite materials was measured on a universal testing machine (858 Mini Bionix; MTS Systems, Eden Prairie, MN, USA) at 23 °C. A defined volume of the various composite paste samples was placed into a glass cylinder (20 mm high × 10 mm diameter) and compacted such that the material completely filled the bottom of the container to a uniform depth. A 7.5 mm diameter brass cylindrical plunger was lowered into the paste at a rate of 5 mm/min until the tip of the plunger was 1 mm from the bottom of the well. The load-extension data were collected and the peak force was recorded. Three measurements were carried out for each material.
2.5
Photopolymerization reaction kinetics and conversion
Real-time monitoring of the polymerization kinetics was carried out using near-infrared (NIR) spectroscopy. The area of the methacrylate CH 2 peak (6163 cm −1 ) was recorded with 4 scans per spectrum at 4 wave-number resolution. To monitor the change of the carbon–carbon double bond concentration during polymerization, a series run collected the predefined peak area as a function of time. Samples ( n = 3) were irradiated for 5 min with the filtered 320–500 nm output of a mercury arc lamp (Acticure 4000; EFOS) at an incident irradiance of 50 mW/cm 2 . Conversion versus time data were plotted and Hill’s 4 parameter non-linear regression was used for curve fitting. As the regression coefficient was greater than 0.99 for all curves, the rate of polymerization ( R p ) was calculated using these data-fitted plots.
2.6
Volumetric shrinkage
A constant volume of each resin was placed onto an aluminum disc in a non-contact linear variable differential transducer-based linometer (Academic Center for Dentistry Amsterdam, The Netherlands). The material was covered with a glass slide, which was adjusted to produce a specimen disc (approximately 1 mm × 6 mm). The displacement caused by linear shrinkage during photopolymerization was measured, and converted to the corresponding volumetric shrinkage. Shrinkage measurements of the dual-filled composite materials were carried out using a mercury dilatometer (Paffenbarger Research Center, American Dental Association, Gaithersburg, MD, USA) with integrated temperature correction. Both the resin and composite specimens were irradiated for 40 s with 400–500 nm light at an irradiance of 600 mW/cm 2 using a halogen dental curing unit (Optilux 501; Demetron Kerr, Orange, CA, USA). The dynamic shrinkage data was recorded during and extending beyond the irradiation interval for a total period of 10 min (resins) or 60 min (composites). Three replicates were carried out for each material.
2.7
Polymerization stress
The dynamic shrinkage stress was evaluated for selected nanogel-modified TEGDMA formulations (0, 20 and 40 wt% reactive nanogel) with a tensometer (Paffenbarger Research Center, American Dental Association) as previously described . A 1 mm × 6 mm specimen size was used with photocuring provided by continuous irradiation by the Acticure mercury arc lamp at 800 mW/cm 2 for 10 min. The simultaneous real-time NIR based conversion data during polymerization was collected in direct transmission mode via fiber optic cables (1 mm diameter single fiber).
2.8
Mechanical strength characterization
Flexural strength tests for all materials were performed using bar specimens ( n = 5) with dimensions of 15 mm × 2 mm × 2 mm photopolymerized between glass slides in an elastomer mold by exposure to halogen-based visible light at 600 mW/cm 2 for 3 min on each side. After 24 h storage in the dark under ambient conditions, the flexural strength ( σ ) and flexural modulus ( E f ) were obtained in three-point bending on the MTS testing machine using a span of 10 mm and a cross-head speed of 1 mm/min.
2.9
Optical properties
The refractive indices of the resins were measured using an Abbe refractometer (model NAR-27; ATAGO Co. Ltd., Tokyo, Japan), at 23 °C with measurement accuracy of ±0.0002. Variations in optical density of the nanogel-loaded monomer and polymer formulations compared with the unmodified TEGDMA monomer and polymer were determined by measurement of the relative transmitted intensities of 758 nm light through specimens ( n = 3) in a 1 cm pathlength cuvette at room temperature.
2.10
Statistical analysis
Data from each evaluation were submitted to one-way analysis of variance (for composites, non-reactive and reactive resins individually), followed by Tukey’s as post hoc test. Comparisons between analogous nanogel concentrations in the reactive and non-reactive subsets were performed by paired t -tests. Regression analyses were carried out to investigate the relationship between nanogel fraction and shrinkage. All analyses were performed at a global level of significance of 95%.
2
Materials and methods
2.1
Nanogel synthesis
Copolymer nanogels were synthesized by a batch process using a 70:30 molar ratio of isobornyl methacrylate (IBMA; Sigma–Aldrich Co., St. Louis, MO, USA) and urethane dimethacrylate (UDMA; Esstech, Essington, PA, USA), respectively. Free-radical polymerization was carried out in solution using a four-fold excess of toluene (relative to monomer mass) as a solvent. Bis(2,4,6-trimethylbenzoyl)-phenylphosphine oxide (BAPO, Irgacure 819; Ciba Specialty Chemicals, Basel, Switzerland) at 2.5 wt% was used as the photoinitiator. 2-Mercaptoethanol (ME; Sigma–Aldrich, 15 mol% relative to monomers) was included as a chain transfer agent to aid in the prevention of macrogelation as well as to provide sites for post-polymerization refunctionalization with methacrylate groups. Room temperature (23 °C) light-activation of the reactant mixture was conducted with an output irradiance of 2500 mW/cm 2 between 320 and 500 nm (Novacure; EXFO, Mississauga, Ontario, Canada) for approximately 2 h during which time, methacrylate conversion of greater than 90% was achieved (based on C C peak area at 1637 cm −1 relative to the C O absorbance at 1720 cm −1 ; includes both pendant vinyl groups and unreacted free monomer) as determined from the initial and final mid-infrared spectra (Nicolet 6700, Thermo Scientific, West Palm Beach, FL, USA). A foil-wrapped 100 mL round-bottom flask was used as the photoreactor with monomer batch sizes of approximately 10 g. Nanogel was precipitated from the clear reaction mixture by dropwise addition into an excess of hexane. The precipitate was filtered and the residual solvent was removed under reduced pressure to obtain the nanogel as a dry powder in approximately 85% yield. In order to obtain reactive, polymerizable nanogels, half of the isolated nanogel was added to a four-fold excess of methylene chloride to give a clear dispersion, which was mixed with a slight excess of 2-isocyanoethyl methacrylate (IEM; Sigma–Aldrich) (equimolar based on the original ME content) and stirred for 6 h in the presence of a trace amount of dibutyltin dilaurate (Sigma–Aldrich) as catalyst. The polymer precipitation method was repeated to isolate the methacrylate-functionalized reactive nanogel. The structures of the reagents used are shown in Fig. 1 .
2.2
Nanogel particle characterization
The polymeric nanogels were characterized by triple detector (differential refractive index, viscosity, light scattering) gel permeation chromatography (GPC; Viscotek, Houston, TX, USA) in tetrahydrofuran using a series of four columns spanning molecular weights of 10 4 –10 7 with absolute molecular weight based on right/low angle light scattering detection calibrated with a 65 kDa poly(methyl methacrylate) standard. As a control, IBMA was homopolymerized in solution with chain transfer agent under the same conditions used to prepare the nanogel. The glass transition temperature ( T g ) of the dry nanogel powder ( n = 2) was determined with a dynamic mechanical analyzer (DMA; Perkin Elmer 8000, Waltham, MA, USA) by sandwiching 10 mg of nanogel in a thin metallic pocket that was then subjected to single cantilever cyclic displacement of 50 μm at 1 Hz. The nanogel was heated to 180 °C with tan δ data collected as the sample was cooled to −50 °C at 2 °C/min in air. The density of the dry nanogel powder was determined using a helium-purged pycnometer (MVP-60C; Quantachrome Instruments, Boynton Beach, FL, USA).
2.3
Formulation of resins and composites
TEGDMA (Esstech) was used as a matrix monomer to be loaded with nanogel. The nanogel was loaded at 5, 10, 20, 30 or 40 wt%, for both reactive and non-reactive particles, with unfilled TEGDMA used as a control. Corresponding dual-filled resin composites were obtained by progressively loading the reactive nanogel-modified TEGDMA samples with the maximum capacity of barium borosilicate glass particles (0.4 μm average diameter) treated with 5 wt% of γ-methacryloxypropyltrimethoxysilane; Esstech) that could be incorporated homogeneously, based on visual inspection coupled with incremental filler addition (single determinations), using a centrifugal mixer (SpeedMixer DAC150; FlackTek, Landrum, SC, USA). In addition, the same TEGDMA/reactive nanogel series was loaded with a uniform barium glass filler content of 70 wt%. In order to render all these materials photo-curable with either UV or visible irradiation, 0.2 wt% of BAPO (relative to the resin content) was included in each mixture.
2.4
Viscosity and consistency
Viscosity measurements of the nanogel-modified TEGDMA materials were performed using a cone-plate digital viscometer (CAP2000+; Brookfield, Middleboro, MA, USA). A defined volume of the control and nanogel-modified monomer was tested at 25 °C under the following conditions: 200 rpm, 1 Hz, and a run time of 30 s. Consistency of the 70 wt% barium glass filled composite materials was measured on a universal testing machine (858 Mini Bionix; MTS Systems, Eden Prairie, MN, USA) at 23 °C. A defined volume of the various composite paste samples was placed into a glass cylinder (20 mm high × 10 mm diameter) and compacted such that the material completely filled the bottom of the container to a uniform depth. A 7.5 mm diameter brass cylindrical plunger was lowered into the paste at a rate of 5 mm/min until the tip of the plunger was 1 mm from the bottom of the well. The load-extension data were collected and the peak force was recorded. Three measurements were carried out for each material.
2.5
Photopolymerization reaction kinetics and conversion
Real-time monitoring of the polymerization kinetics was carried out using near-infrared (NIR) spectroscopy. The area of the methacrylate CH 2 peak (6163 cm −1 ) was recorded with 4 scans per spectrum at 4 wave-number resolution. To monitor the change of the carbon–carbon double bond concentration during polymerization, a series run collected the predefined peak area as a function of time. Samples ( n = 3) were irradiated for 5 min with the filtered 320–500 nm output of a mercury arc lamp (Acticure 4000; EFOS) at an incident irradiance of 50 mW/cm 2 . Conversion versus time data were plotted and Hill’s 4 parameter non-linear regression was used for curve fitting. As the regression coefficient was greater than 0.99 for all curves, the rate of polymerization ( R p ) was calculated using these data-fitted plots.
2.6
Volumetric shrinkage
A constant volume of each resin was placed onto an aluminum disc in a non-contact linear variable differential transducer-based linometer (Academic Center for Dentistry Amsterdam, The Netherlands). The material was covered with a glass slide, which was adjusted to produce a specimen disc (approximately 1 mm × 6 mm). The displacement caused by linear shrinkage during photopolymerization was measured, and converted to the corresponding volumetric shrinkage. Shrinkage measurements of the dual-filled composite materials were carried out using a mercury dilatometer (Paffenbarger Research Center, American Dental Association, Gaithersburg, MD, USA) with integrated temperature correction. Both the resin and composite specimens were irradiated for 40 s with 400–500 nm light at an irradiance of 600 mW/cm 2 using a halogen dental curing unit (Optilux 501; Demetron Kerr, Orange, CA, USA). The dynamic shrinkage data was recorded during and extending beyond the irradiation interval for a total period of 10 min (resins) or 60 min (composites). Three replicates were carried out for each material.
2.7
Polymerization stress
The dynamic shrinkage stress was evaluated for selected nanogel-modified TEGDMA formulations (0, 20 and 40 wt% reactive nanogel) with a tensometer (Paffenbarger Research Center, American Dental Association) as previously described . A 1 mm × 6 mm specimen size was used with photocuring provided by continuous irradiation by the Acticure mercury arc lamp at 800 mW/cm 2 for 10 min. The simultaneous real-time NIR based conversion data during polymerization was collected in direct transmission mode via fiber optic cables (1 mm diameter single fiber).
2.8
Mechanical strength characterization
Flexural strength tests for all materials were performed using bar specimens ( n = 5) with dimensions of 15 mm × 2 mm × 2 mm photopolymerized between glass slides in an elastomer mold by exposure to halogen-based visible light at 600 mW/cm 2 for 3 min on each side. After 24 h storage in the dark under ambient conditions, the flexural strength ( σ ) and flexural modulus ( E f ) were obtained in three-point bending on the MTS testing machine using a span of 10 mm and a cross-head speed of 1 mm/min.
2.9
Optical properties
The refractive indices of the resins were measured using an Abbe refractometer (model NAR-27; ATAGO Co. Ltd., Tokyo, Japan), at 23 °C with measurement accuracy of ±0.0002. Variations in optical density of the nanogel-loaded monomer and polymer formulations compared with the unmodified TEGDMA monomer and polymer were determined by measurement of the relative transmitted intensities of 758 nm light through specimens ( n = 3) in a 1 cm pathlength cuvette at room temperature.
2.10
Statistical analysis
Data from each evaluation were submitted to one-way analysis of variance (for composites, non-reactive and reactive resins individually), followed by Tukey’s as post hoc test. Comparisons between analogous nanogel concentrations in the reactive and non-reactive subsets were performed by paired t -tests. Regression analyses were carried out to investigate the relationship between nanogel fraction and shrinkage. All analyses were performed at a global level of significance of 95%.
3
Results and discussion
3.1
Nanogel synthesis
The effects of variations in the selection and relative concentrations of monomers, chain transfer agents, initiators and solvent, as well as the in the choice of reaction conditions, on nanogel structure and properties will be addressed in a separate report. Here, we have elected to highlight one basic nanogel material, in both reactive and non-reactive forms, as an additive in TEGDMA monomer and in analogous composite formulations prepared with barium glass filler. An UV/vis light-induced solution polymerization was used to generate the nanogel material with high efficiency. Under the conditions used here, a chain transfer agent is not necessary to avoid macrogelation, even at high conversion and with the relatively high concentration (30 mol%) of UDMA used as the crosslinker. However, inclusion of an alkyl thiol limits polymer chain length and introduces a relatively high concentration of chain ends, many of which are subsequently appended with a reactive urethane methacrylate group from the addition of IEM to the hydroxyl group associated with ME located nearly exclusively at chain ends based on the chain transfer process. The mid-IR spectra obtained from the initial and final nanogel reaction mixture indicates a conversion of 92%, which increases to approximately 95% conversion for the precipitated nanogel where the residual methacrylate represents the limited number of pendant double bonds within the nanogel. The mid-IR analysis is also useful to follow the addition of IEM to the thiol-terminated chain ends. The location of methacrylate groups on mobile and readily accessible chain ends may make these reactive sites more effective in copolymerization with the matrix monomer than the pendant vinyls remaining from the initial nanogel synthesis. The consumption of alkyl thiol and methacrylate monomer in chain transfer dominated network photopolymerizations has been described previously . Based on the thiol absorbance at 2585 cm −1 in the mid-IR spectra, it appears that only about 25% of the ME is incorporated into the nanogel; however, the IR also shows that the nanogel precipitation step effectively removes the excess thiol and there is no thiol odor associated with the precipitated nanogel. Our prior work indicated the mechanical property advantages associated with the use of reactive nanogels, rather than the essentially inert initially prepared nanogels, to modify dental resins .
3.2
Particle characterization
Triple detection GPC analysis of the nanogels provides not only molecular weight and polydispersity data, but also information on molecular structure and size based on the viscosity and light scattering detection capabilities along with the standard refractive index mode of detection. The roughly spherical or globular structure of the nanogel is confirmed by the Mark–Houwink α value of 0.37 as opposed to a typical value of approximately 0.7 associated with a random coil linear polymer structures . The IBMA solution homopolymerization in the presence of ME produced polymer with a weight average molecular weight ( M w ) of 5600 Da and a polydispersity of 1.3. With UDMA included in the polymerization, nanogel is formed with a M w of 1.02 × 10 6 Da, a polydispersity of 5.8 and an average hydrodynamic radius of 8.6 nm with an upper limit of 30–40 nm. By application of the IBMA homopolymer as a linear reference and assuming a trifunctional branching model in the Zimm–Stockmayer expression , the GPC-based calculated weight average branching number per nanogel is approximately 60, which indicates a highly branched and cyclized macromolecular structure.
The thermal scan of the bulk nanogel powder by dynamic mechanical analysis provided a T g of 92.9 ± 0.8 °C and a tan δ peak width at half height of 32 ± 4 °C. As a control, a commercial poly(methyl methacrylate) (PMMA; Sigma–Aldrich; 120,000 Da) powder was evaluated in the DMA under the same conditions with the resulting T g value of 108.8 ± 0.7 °C in reasonable agreement with the PMMA literature value of 105 °C . The nanogel T g is significantly lower than that of IBMA homopolymer (145.3 ± 2.2 °C with a half-height tan δ peak width of 54.1 ± 0.4 °C) probably as a result of the relatively high concentration of chain ends associated with the highly branched but open nanogel structure; however, the nanogel T g is significantly higher than that of TEGDMA homopolymer photocured at room temperature. The density of the collapsed nanogel as an aggregated dry powder was approximately 1.14 g/cm 3 . This value is bracketed by the densities of TEGDMA monomer and homopolymer (70% conversion) at 1.07 and 1.18 g/cm 3 , respectively. The barium glass filler used in the composite samples had a density of 3.04 g/cm 3 and therefore, the 70 wt% inorganic filler loading accounts for volume fractions of 45.1% in the TEGDMA control and 45.7% in the experimental material at the 40 wt% nanogel loading level (assuming additive densities within the resin phase).
3.3
Monomer viscosity, filler loading capacity and composite consistency
The effect of low nanogel additive contents on TEGDMA viscosity ( Table 1 and Fig. 2 ) is minimal as is typically observed for globular/spherical hyperbranched or dendritic polymeric additives . While the difference was small, the reactive nanogel bearing urethane methacrylate functionality consistently yielded lower viscosity TEGDMA compositions compared with the same mass fraction of the inert nanogel with the stronger hydrogen bonding hydroxyl functionality . Irrespective of whether reactive or non-reactive nanogels were employed, as the nanogel loading levels extend beyond 20 wt%, the percolation threshold is approached where particle–particle interactions begin to dominate over resin–particle interactions. A calculation based on monodisperse 10 nm nonporous particles predicts approximately 5 nm inter-particle spacing at only a 20 vol% loading level . Here, the nanogel particles are significantly swollen with monomer, which leads to an increased effective volume fraction compared with the calculation based simply on mass and density considerations. The exponential rise in viscosity at high nanogel content is an indication that at 40 wt% nanogel, the matrix phase is approaching a continuous interphase rather than some combination of bulk TEGDMA and the interfacial regions around the nanogels. However, as shown in Table 1 , despite the presumptive limitation of a bulk TEGDMA phase at high nanogel concentrations, the maximum loading of inorganic filler was only modestly reduced compared with the TEGDMA control.
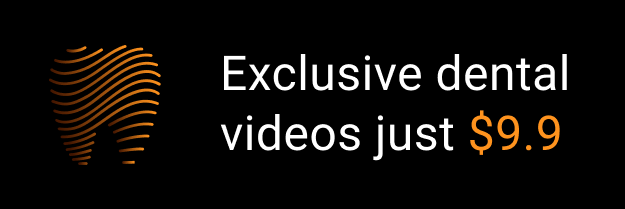