8
Contemporary Periodontal Regenerative Treatment
Ronaldo Barcellos de Santana, Mehmet Ilhan Uzel, and Carolina Miller Mattos de Santana
Despite the successful clinical results, conventional therapeutic procedures present significant variability depending on the intrinsic healing potential of the patient, which are typically observed as long junctional epithelium and limited regeneration of the periodontal attachment apparatus due to minimal cementogenesis and limited bone formation (Listgarten and Rosenberg 1979; Steiner et al. 1981; Waerhaug 1978). Ideally, the healing process should reestablish the original tissue architecture and function of the support tissues previously destroyed, including the regeneration of alveolar bone, radicular cementum, and periodontal ligament (Caton and Greenstein 1993; Listgarten and Rosenberg 1979; Mellonig 1999). Therefore, several procedures have been employed to optimize the regenerative potential of periodontal lesions, including bone grafts and substitutes, barrier membranes for guided tissue regeneration, bioactive substances such as growth factors and enamel matrix derivatives and combination of these techniques and materials (Pretzl et al. 2009; Sanz et al. 2004, Siciliano et al. 2011; Thakare and Deo 2012; Yukna et al. 2002).
In conjunction with, and irrespective of, the selection of an adequate regenerative approach, the use of a sound treatment protocol and surgical technique is mandatory to optimize the clinical results including the flap design, treatment of the root surface, treatment of the bone defect, maintenance and control and timing of reevaluation.
Flap Design
Adequate flap design is an important element in periodontal regenerative therapy in order to provide adequate access for root instrumentation, bone defect debridement, placement of regenerative materials in the periodontal defect, and attaining primary coverage of the surgical site. Several types of flaps have been used, including the modified Widman flap (Cortellini et al. 1995; de Miranda et al 2013; Santana et al 1999), the papilla preservation flap (Takei et al. 1989), modified papilla preservation flap (Cortellini et al. 1995; Santana and de Santana 2015), simplified papilla preservation flap (Cortellini et al 1999), interproximal tissue maintenance (Murphy 1996), and single flap approaches (Trombelli et al. 2012). Traditionally, conventional flap designs such as the modified Widman flap were employed for the treatment of intrabony (Cortellini et al. 1999) and furcation defects (de Miranda et al. 2013; Santana and Van Dyke 1999); however, conventional surgical approaches performed with vertical releasing incisions and without interdental papilla preservation may result in deficient flap closure, limited blood clot stability and, possibly, negatively impacting periodontal wound healing. Thus, the necessity to obtain and maintain adequate levels of soft tissue coverage post-surgery led to the increased test and development of alternative approaches.
The coronally positioned flap was proposed as a method to obtain coverage of regenerative materials and primary closure of the surgical site; however, limited additional benefit in probing depth reduction and attachment level gain was provided after the use of this flap design in the treatment of human furcation defects, in comparison with the modified Widman flap, except for significantly reduced postsurgical gingival recession (Santana and Van Dyke 1999). However, positive outcomes were reported for this procedure when used in conjunction with guided tissue regeneration and bone substitutes (Santana and Van Dyke 1999, Santana et al. 2009).
In order to optimize the healing potential of human intrabony defects, several technical modifications were proposed in order to favor the maintenance of the interproximal soft tissue coverage flap closure (Cortellini et al. 1995; 1999; Murphy 1996; Takei et al. 1989; Trombelli et al. 2012). The preservation of the interproximal soft tissues may result in higher blood clot stability within the periodontal defect and faster vascular stability in the papillary area (Retzepi et al. 2007), promoting primary wound healing, improved wound instability and, ultimately, enhanced clinical results in the treatment of human intrabony defects (Cortellini and Tonetti 2007a, 2007b; Cortellini et al. 2008; Retzepi et al. 2007). Increased reduction in probing depth and attachment levels with minimal gingival recession and less postoperative discomfort has been reported (Graziani et al. 2012).
Bone Grafts and Bone Substitutes
Bone grafts and bone substitutes are biocompatible particulate biomaterials that are placed directly into periodontal bone defects to serve as scaffolds for cell attachment, migration, and differentiation.
Several bone grafts/substitutes have been used in the regenerative treatment of periodontal defects, including autografts, allografts, xenografts, and alloplastic materials (Sculean et al. 2015). For many years, bone autografts were considered the gold standard for bone regeneration due to its potential osteogenic, osteoconductive, and osteoinductive potentials. Clinical evidence, however, did not demonstrate significantly enhanced clinical results in comparison to periodontal open flap debridement procedures without the use of bone grafting (Froum et al. 1976; Renvert et al. 1985). Histological evidence in humans is controversial, with some studies demonstrating periodontal regeneration (Dragoo & Sullivan 1973a, 1973b; Hiatt et al. 1978; Stahl et al. 1983), while others demonstrated the formation of a long junctional epithelium (Listgarten and Rosenberg 1979), particularly for bone grafts harvested from intraoral sources. Moreover, complications such as ankylosis and root resorption were also reported, particularly following the use of bone allografts from extra-oral sites, such as medullary bone from the iliac crest (Dragoo and Sullivan 1973a, 1973b). Taken together, the current evidence may limit the indication of bone autografts for the treatment of periodontal bone defects.
Bone allografts may contain bone morphogenetic proteins, providing these materials with osteoinductive activities (Miron et al. 2016; Schwartz et al. 1996, 1998). However, the osteoinductive activity of bone allografts such as DFDBA varies significantly depending on the manufacturer or the gender of the donor (Schwartz et al. 1996, 1998). Thus, adequate donor selection and manufacturing processes that preserve the bioactivity of the material appear to be critical for the production and clinical use of these materials (Schwartz et al. 1996, 1998). In conjunction with adequate clinical (Barnett et al. 1989; Flemmig et al. 1998; Meadows et al. 1993; Rummelhart et al. 1989) and histologic results (Bowers et al. 1989a, 1989b), robust histologic evidence of periodontal regeneration in humans were reported (Bowers et al. 1989a, 1989b) following the use of these materials.
Several xenogeneic materials, derived from bovine, swine, or equine sources, have also been employed, and act as an inert bone scaffold of tridimensional structure like the mineralized bone matrix. It has been hypothesized that these materials could present the risk of antigenicity, being derived from other species (Oliveira et al. 2008) and, despite controversial, the risk for prion infection has also being considered (Kim et al. 2013, 2016). Adequate clinical (Figures 8.10 to 8.17) results were reported for these materials in the treatment of periodontal defects (Camargo et al. 2000; Cosyn et al. 2012; De Bruyckere et al. 2018; Pietruska 2001; Richardson et al 1999; Scheyer et al. 2002; Sculean et al. 2002b).

Figure 8.10 Baseline clinical (A) and radiographic view (B). Deep probing depths, bleeding on probing (A), and vertical radiographic bone radiolucency (B) were noted on the mesial aspect of the maxillary second premolar.

Figure 8.11 Trans surgical view after full-thickness flap reflection and mechanical root treatment (A to C) revealed deep intrabony defect on the mesial and palatal aspects of the maxillary second premolar.

Figure 8.12 Following mechanical instrumentation, the roots were chemically conditioned for 3 min with a 24% EDTA gel (A and B), and then abundantly washed with a saline solution.

Figure 8.13 An EMD gel (Emdogain®) was applied over the root surfaces (A) and into the bone defect (B).

Figure 8.14 A particulate bovine xenograft was preconditioned with EMD and carefully packed into the bone defect.

Figure 8.15 A new layer of EMD gel was applied over the grafted area (A) and root surfaces (B).

Figure 8.16 The flap was coronally advanced and sutured with interrupted sutures.

Figure 8.17 Clinical view one year after treatment demonstrated clinically health gingival tissues, absence of bleeding on probing and 2.5 mm probing depth (A). Radiographic evaluation performed five years after treatment revealed significantly increased radiopacity on the mesial aspect of the treated second premolar (B).
Systematic analysis of histologic preclinical essays, in which regenerative therapies were used for the treatment of periodontal defects (Ivanovic et al. 2014), demonstrated that most of the biomaterials, used alone or combined, promoted more extensive periodontal regeneration than conventional open flap procedures without the use of biomaterials (Ivanovic et al. 2014). Systematic reviews also concluded that the use of bone grafts/substitutes resulted in significant enhancement in attachment levels, probing depth reduction, increased bone fill, and reduced crestal resorption in comparison with open flap debridement (Reynolds 2003). However, attachment level gains varied significantly among the several individual biomaterials (Trombelli 2002). Similar clinical results were obtained for allografts and alloplastic materials (Barnett et al. 1989; Bowen et al. 1989; Oreamuno et al. 1990). The combined use of bone grafts and membranes can result in increased gains of attachment, bigger probing depth reduction and more defect filling than membranes used alone (Murphy and Gunsolley 2003; Needleman et al. 2006). It has been reported that the use of alloplastic materials may reduce postsurgical gingival recession (Leknes et al. 2009), possibly by avoiding the collapse of the soft tissues into the bone defects. Histologically, DFDBA, autografts, and some xenografts result in the formation of new attachment and periodontal regeneration (Sculean et al. 2003a; Sculean et al. 2003b; Sculean et al. 2005b; Sculean et al. 2015), while the results for alloplastic materials are more limited, specially, when employed in monotherapy (Sculean et al. 2015). However, some alloplastic materials can result in periodontal regeneration when employed in conjunction with enamel matrix derivatives (Sculean et al. 2008c).
Guided Tissue Regeneration
Guided tissue regeneration is a treatment concept based on the use of membranes or barriers interposed between the surgical flap and the periodontal defect to mechanically exclude non-regenerative cells from the gingival epithelium and connective tissue from the regenerative events at the defect area (Figures 8.1 to 8.9). The modern biological concepts of guided tissue regeneration (GTR) is based in the establishment of a protected environment for the blood clot and competent regenerative cells via the interposition of a physical barrier between the soft tissues of the gingival flap and the osseous defect/root surfaces in order to isolate a secluded space, thus, allowing cells from the periodontal ligament and bone to recolonize the defect area and regenerate the periodontal attachment apparatus (Caffesse et al. 1988; 1990; Gottlow et al. 1986; Magnusson et al. 1988; Nyman et al. 1982; Pitaru et al. 1987; Pfeifer et al. 1989). These concepts have been used clinically for the treatment of several periodontal and bone deformities such as periodontal intrabony (Cortellini and Tonetti 2000), furcation (Santana and Van Dyke 1999; Santana et al. 2009) (Figures 8.6 to 8.10), and recession defects (Trombelli and Calura G 1993); closure of oro-antral communications (Waldrop and Semba 1993); surgical treatment of periodontal defects associated with palato-gingival developmental grooves (Rankow and Kassner 1996); reconstruction of bone defects following surgical removal of oral cysts or tumors (Vitkus and Meltzer 1996); autogenous tooth transplantation (Hurzeler and Quinones 1993); surgical treatment of advanced apical or combined apical-marginal lesions associated with absence of buccal alveolar bone wall (Rankow and Kassner 1996, Santana and Santana 2013) (Figures 8.1 to 8.5); maintenance or enhancement of the osseous ridge, following root amputation of multi-rooted teeth (Conner 1996); and prevention or treatment of distal osseous defects following the removal of impacted third molars (Oxford et al. 1997).

Figure 8.1 Clinical trans-surgical view. A significant bucco-palatal through-and-through maxillary bone defect can be observed extending apically up to the nasal floor. Santana et al., 2013 / Reproduced with permission from John Wiley & Sons .

Figure 8.2 Radiographic view at baseline. Santana et al., 2013 / Reproduced with permission from John Wiley & Sons.

Figure 8.3 Area was grafted with microparticulate hydroxyapaptite and the surgical site was covered with PTFE membranes after root management and apical retrofilling. The flap was coronally advanced and stabilized with interrupted sutures. Santana et al., 2013 / Reproduced with permission from John Wiley & Sons.

Figure 8.4 Trans-surgical view at the time of membrane removal nine months after the surgical procedure (A) and radiographic view after 15 years of healing demonstrates significant and stable periodontal healing and bone fill. Santana et al., 2013 / Reproduced with permission from John Wiley & Sons.

Figure 8.5 Baseline clinical (A) and radiographic view (B). A buccal class II furcation involvement was detected on the mandibular left first molar.

Figure 8.6 Trans surgical view after full-thickness flap reflection and mechanical root treatment (A) revealed 3.5 mm vertical (B) and 7 mm horizontal (C) buccal interradicular bone loss.

Figure 8.7 A synthetic polylactic acid barrier membrane was adjusted and sutured over the defect (A) and the gingival flap was released, coronally advanced, and sutured with suspensory sutures (B).

Figure 8.8 Clinical view one year after treatment demonstrated clinically health gingival tissues (A), absence of bleeding on probing, and 2.5 mm probing depth (B).

Figure 8.9 Radiographic evaluation performed five years after treatment revealed significantly increased radiopacity on the furcation of the treated first molar, however, the second molar exhibited clinical and radiographic signs of vertical mesial root fracture (A), which could be confirmed after the tooth was extracted (B) during surgical bone reconstructive approach for implant placement (C). The furcation area of the treated first molar, however, was completely closed with new bone (C).
Several materials have been tested as barrier membranes for GTR. These materials exhibit differences in chemical composition, physical properties, and macroscopic and microscopic structure. Criteria for the design of GTR membranes have been proposed (Scanttlebury 1993), and include tissue integration capacity, cell occlusivity properties, clinical manageability, space making ability, and biocompatibility. The biological response to implanted biomaterials is a critical element in reconstructive biology. Significant differences have been described for the response of living tissues to implanted barrier membranes, possibly related to the surface topography and porosity of the device (Buchmann et al. 2001; Lu et al. 2004; Lundgren et al. 1995; Scanttlebury et al 1993; Santana et al. 2010; Wikesjo et al. 2003).
Nonabsorbable Devices
Polytetrafluorethilene (PTFE) is an inert and biocompatible material. Due to a unique molecular composition and configuration PTFE presents with low chemical reactivity, great stability, and low superficial energy of the polymer. There is no solvent that can dissolve PTFE in temperatures lower than 3,000°C. PTFE can be utilized as barrier devices in porous, dense, and expanded forms (Crump et al. 1996; Scattlebury 1993). Other materials tested as nonabsorbable barriers include microfibrillar alkali-cellulose, aluminum, and titanium. Microfibrillar alkali-cellulose is a safe and biocompatible material, primarily used as a skin substitute for burned people (Novaes Jr. et al 1990a, 1990b, 1992). A core of commercially-pure aluminum (minimum 99.35% of Al), superficially coated with aluminum oxide (minimum of 99.48% of Al2O3), in a laminated form, Al2O3 is a ceramic material which has biocompatibility characteristics similar to titanium and tantalus oxides (TiO2, Ta2O5) that has been used as constituents of endosseous dental implants, both in single-crystal and polycrystalline forms (Babbush et al. 1987; Kawahara and Hirabayashi 1980). Biocompatibility of this material appears to be derived from its high dielectric constant, biomolecule adsorption capacity, chemical inertness to corrosion, low dielectric potential difference, and catalyst activity (Kawahara and Hirabayashi 1980; Yamagami et al. 1988). Among the several alternatives for nonabsorbable devices, PTFE is the most studied and well characterized.
Synthetic Absorbable Devices
Several synthetic materials have been tested as resorbable GTR membranes. Calcium sulfate is one of the materials that have been tested as a barrier for GTR applications (Conner 1996; Sottosanti 1992, 1993, 1995). Although reintroduced in the 90s, similar materials have been used for more than 100 years in medicine and dentistry as adjuncts for bone regeneration (Peltier 1959; Shaffer and App 1971). At present, it is composed of absorbable medical grade calcium sulfate monohydrate (CaSO4), plaster of Paris, mixed with a premeasured accelerator solution. It is used as a barrier over autogenous or allogenous bone grafts, in order to maintain the graft in place. Spagnuolo and Bissada (1995) proposed a new resorbable composite barrier for the treatment of adult periodontitis membrane composed of calcium sulfate monohydrate (CaSO4) combined to DFDBA and doxycycline in a 4:1:1 ratio by volume.
Polyglactin 910 is a lactide-glicolide copolymer which has been long used as a resorbable suture material (Conn et al. 1974). Such material seemed to be inert, non-antigenic, and non-pyogenic, presenting only a mild tissue reaction during absorption (Conn et al. 1974). It has been manufactured as a mesh for use in neurosurgery (Maurer and McDonald 1985) and is composed of undyed fiber, identical in composition to that of the absorbable sutures. This mesh has also been tested for GTR procedures (Cristgau et al. 1998; Eikholtz and Hausman 1998; Fleisher et al 1988).
A synthetic, liquid polymer of lactic acid, poly(DL-lactide), dissolved in N-methyl-2-pyrrolidone(NMP), has been developed for GTR purposes (Polson et al. 1994, 1995).This material exists as a fluid which transforms to a solid on contact with water or other aqueous solutions. This property is used to form an adaptable partially set barrier extraorally, which is rigid enough to provide GTR function but flexible enough to be easily adapted to the surgical site. The barrier continues to solidify in situ and subsequently bioabsorbs via hydrolysis (Garrett et al 1997). It has a similar composition, rate of absorption, and safety performance as that of Polyglactin 910. It has been shown to be safe, nontoxic, resorbable, and efficacious for GTR attempts in animals and humans (Garrett et al. 1997; Polson et al. 1994, 1995).
Various other polylactic acid-based products have been evaluated for their potential use in GTR surgeries, such as (a) polylactic acid dissolved in chloroform (Magnusson et al. 1988), (b) polylactic acid blended to a citric acid ester (Gottlow 1993), and (c) a polylactic acid porous sheet (Vernino et al. 1995). Such materials are absorbed by hydrolysis and seem to break down with minimal inflammatory reaction (Vernino et al. 1995), which seems to be consistent with a material that was biologically acceptable in the wound healing environment (Vernino et al. 1995). A hydrophilic, biodegradable membrane fabricated from D,L-polylactic acid was introduced (Vernino et al. 1998, 1999). The thickness of this membrane measures from 175 to 250 μm, and the polymer of the membrane is organized in an architecture of randomly sized, randomly positioned, intercommunicating interstices ranging in size from 10 to 15 μm. One surface of the membrane, constituting approximately 10% of the total thickness, is considerably denser than the remainder of the device (Vernino et al. 1998). Another of these polylactic acid-based products is a bioabsorbable material consisting of polylactic acid comprising L-and D-lactic acid enantiomers, and acetyl-tributyl citrate, a citric acid ester. It has a multilayered matrix designed for ingrowth of gingival connective tissue. Periodontal ligament and alveolar bone can also migrate into the matrix and merge with the gingiva. Thus, the matrix barrier would allow for simultaneous regeneration and integration following a single surgical procedure (Gottlow 1993). Clinical results were reported for the treatment of human gingival recession, furcation, and intraosseous lesions with this material (Cristgau et al. 1998; Luepke et al. 1997; Pini Prato et al. 1995).
Organic Absorbable Devices
Among the products of organic or natural sources, collagen-based devices of different types and origins are probably the most commonly used as barrier membranes due to its weak immunogenic capacity and long-term experimental use in animals and humans (Pitaru et al. 1988). Several characteristics of collagen seem to support its use as barrier membranes for GTR purposes (Pitaru et al. 1988). Collagen is the major extracellular macromolecule of the periodontal connective tissues (Posthlethwaite et al 1978) that can act as a barrier for migrating gingival epithelial cells (Pitaru et al. 1987) by providing a thrombogenic surface (Blumenthal 1993) that inhibits the mitotic function of the basal epithelial cells during the initial phases of wound healing (Numabe et al. 1993), thus limiting the apical migration of epithelium. Simultaneously, the hemostatic properties of collagen (Pitaru et al. 1988) may enhance blood clot stability in the bony defect, while exhibiting a chemotactic activity for fibroblasts (Posthlewaite et al. 1978). Despite the past controversies regarding the safety of using collagenous membranes in human clinical trials, due to localized hypersensitivity reactions developed in sites where collagen was injected (Kammer and Chukurian 1984), collagenous membranes did not elicit any abnormal immunologic responses when implanted as a barrier membrane during GTR attempts in humans (Black et al. 1994; Blumenthal 1993; Mattson et al. 1995; Numabe et al. 1993).
Autogenous “periosteum” grafts were proposed by Lekovic et al. (1991) as an alternative for GTR barriers. In this technique, a partial thickness connective tissue graft is obtained from the palate of the patient, then trimmed and adjusted as a barrier to cover the periodontal defect to be treated according to the principles of GTR. In controlled studies, this technique demonstrated significantly better results than the controls treated by a modified Widman flap procedure (Lekovic et al. 1991), and similar results to those obtained by ePTFE barriers (Bouchard et al. 1993) in the treatment of human mandibular furcation lesions. However, the necessity of a second surgical site to obtain the graft, the limited and great variability of the results obtained with this technique diminished its applicability in routine regenerative periodontal therapy.
Particulate decalcified freeze-dried bone allografts (DFDBA) have been used for many years as a periodontal grafting material (Gouldin et al. 1996; Mellado et al. 1995; Mellonig 1984; Pearson et al. 1981; Quintero et al. 1982; Wallace et al. 1994) due to the safety, biocompatibility, and very weak immunogenic properties of these materials (Friedlaeder et al 1984; Quattlebaum et al. 1988; Turner and Mellonig 1981). Moreover, possible osteoinductive capacity, due to the presence of bone morphogenetic proteins (BMPs), may be retained in the material following its processing (Urist 1965; Urist and Dowell 1968; Urist et al. 1967), promoting bone inductive abilities in vivo (Mellonig et al. 1981a, 1982, 1981b, 1983). Despite the demonstration of biological activity of DFDBA (Shigeyama et al. 1995), such activity could be variable, depending on the source of the product, donor characteristics, and the processing of the material (Schwartz et al. 1996). Thus, the effectiveness of particulate DFDBA on periodontal regeneration has been questioned (Becker et al. 1994, 1995). Laminar sheets of DFDBA are available and have been successfully employed as barriers for GTR (Rankow and Kassner 1996; Scott et al. 1997; Yamaoka et al. 1996). Positive results were reported in the treatment of human furcation defects (Scott et al. 1997; Yamaoka et al. 1996).
Bioabsorbable composite matrixes were also tested as a barrier for clinical use in GTR applications (Costa-Noble et al 1996). The basic components of this material are elastin and fibrin monomers that are combined under specific physicochemical conditions. The crude product obtained is then further processed with fibronectin, type I collagen, and other aggregating agents as sulfur derivatives and gamma-rays to obtain optimum cohesivity. The derived material is also associated with polyglactic networks to obtain good saturability and treated with aprotinin to control the degradation rate.
Histologic preclinical studies demonstrated that barrier membranes used alone or combined with bone grafts/substitutes result in bone (Santana et al. 2010) and periodontal regeneration (Sculean et al. 2008c). Enhanced results were observed for the combined therapies employing membranes and bone grafts/substitutes in comparison to the use of membranes alone in noncontained two-wall defects and supra-alveolar defects, while no additional benefit was observed for the combined therapy for three-wall defects (Sculean et al. 2008c). Results from histological studies in humans are partially divergent than these results obtained in animals.
The results of clinical studies and systematic reviews demonstrated that regenerative therapies with barrier membranes provide significant clinical benefits, in comparison with open flap procedures alone, as evaluated by reduced gingival recession, probing depth reduction, attachment level gain, and bone fill (Cortellini and Tonetti 2000; Murphy and Gunsolley 2003; Needleman et al. 2005). Clinical results may be maintained for several years in most patients enrolled in frequent periodontal supportive therapy, even with severe intrabony defects (Cortellini et al 2004). Positive outcomes have also been reported for the combined use of membranes and synthetic graft materials in the treatment of human furcation defects (Santana and Van Dyke 1999; Santana et al. 2009). No significant difference was reported for the several types of membranes evaluated; however, the use of different types of membranes may explain the significant variability of clinical results reported in different studies (Murphy and Gunsolley 2003; Needleman 2006).
Bioactive Substances
The use of bioactive substances offers a new paradigm in periodontal reconstructive therapy as a resource to amplify or accelerate the endogenous healing potential with new therapies that act at the cellular and molecular levels to enhance the periodontal wound healing and regeneration of the attachment apparatus.
Autogenous Platelet Concentrates
Autogenous platelet concentrates preparations have been widely tested as regenerative biomaterials in periodontics. The main goal of these preparations is to obtain concentrate supraphysiological doses of autologous growth factors from centrifugated blood (Kobayashi et al. 2016). Several types of platelet concentrates have been described, depending on the centrifugation protocol employed (Castro et al. 2019; Choukroun 2015; Choukroun et al. 2001; Cortellini et al. 2018; Miron et al. 2019a, 2019b, 2017; Wend et al. 2017). Platelet rich plasma (PRP) is a platelet concentrate produced using anticoagulants and high g-forces to selectively separate blood cells based on density, under the centrifugation cycles ranging from 15 min to 1 h, depending on the specific harvesting kits employed. PRP protocols traditionally result in the production of three layers: the upper or platelet-poor (acellular) layer, the middle platelet-rich (buffy coat) layer, and a bottom red blood cell (RBC corpuscle) layer. Since anticoagulants are utilized, the cell layers are separated without coagulating; however, it has been also reported that anticoagulant incorporation in formulations may negatively impact tissue regeneration (Anfossi et al. 1989; Fijnheer et al. 1990; Marx 2004).
Platelet-rich fibrin (PRF) was developed as a second-generation platelet concentrate with the aim of eliminating the need for anticoagulants (Choukroun et al. 2001). In this protocol, the centrifugation times were significantly reduced to only 8–12 min. An important modification of the PRF protocol, denominated advanced PRF (A-PRF), was the decreased centrifugation speeds from 2,700 rpm to 1,300 rpm, resulting in a PRF formulation with a higher number of leukocytes which were also more evenly distributed throughout the blood clot (Choukroun 2015; Miron et al. 2019a). This preparation demonstrated higher growth factor release and increased fibroblast migration, proliferation, and collagen mRNA levels than the traditional PRF (Fujioka-Kobayashi et al. 2017; Kobayashi et al. 2016).
The injectable PRF (iPRF) is a variation of the protocol that was developed by further reducing centrifugation speed and time and by using centrifugation tubes with more hydrophobic materials to reduce clotting times (Miron et al. 2017). Comparatively to PRP protocols, the iPRF protocol results in a low yield of isolated platelets and leukocytes (Miron et al. 2019b; Varela et al 2019), which demonstrate significantly increased cellular activity (Abd El Raouf et al. 2017; Miron et al. 2017; Wang et al. 2017, 2018). However, the total platelet yield and growth factor release is higher on PRP preparations (Miron et al. 2017). More recently, in order to improve the platelet and leukocyte yields of i-PRF, a novel protocol, termed concentrated PRF (C-PRF), has been developed (Castro et al. 2019; Cortellini et al. 2018; Miron et al. 2019b; Wend et al. 2017). In this novel protocol, centrifugation times are increased to 4–8 min, centrifugation speeds are increased to 2,700–3,000 rpm, and a horizontal centrifugation (as opposed to conventional fixed angle centrifugation) is employed (Castro et al. 2019; Cortellini et al. 2018; Miron et al. 2019b; Wend et al. 2017). These modifications resulted in increased platelets accumulation in the upper i-PRF layer (Wend et al. 2017), and higher platelet and leukocyte yields and concentrations (Castro et al. 2019; Cortellini et al. 2018; Miron et al. 2019b). Interestingly, a study that employed a modified L-PRF protocol (2,700 rpm for 12 min) found that the top 4 mL layer was completely devoid of cells, while the majority of cells, amounting to tenfold the cell counts at baseline, were found within the buffy coat layer immediately above the RBC corpuscle layer (Miron 2019b). These cells derived from the buffy coat may secrete up to threefold the amount of growth factors release (PDGF-AA, TGF-B1, and EGF) in comparison to the conventional iPRF protocol (Fujioka-Kobayashi et al. 2020). The PRF obtained from this harvesting technique was named concentrated PRF (C-PRF) and this preparation may exhibit higher growth factor release as well as superior cellular activity.
Data from a recent systematic review of randomized clinical studies demonstrated that the use of PRF in conjunction with open flap debridement resulted in significant improvements in probing depth reduction, attachment level gains, and bone fill (Miron et al. 2021). However, when PRF was used in conjunction with or versus bone grafts/substitutes most of the studies included in the review failed to demonstrate additional benefits of PRF in probing depth reduction or attachment level gain (Miron et al. 2021).
Enamel Matrix Derivative
Amelogenins are a heterogeneous group of low molecular weight proteins which are the most prevalent (>90%) component of the organic fraction of enamel (Esposito et al. 2004). These proteins promote the adhesion and proliferation of periodontal ligament cells, increased secretion of growth factors (TGF-1, PDGF-AB, IGF-I), production of extracellular matrix (hyalurans, proteoglycans, versican, biglycan and decorin), and mineralization (Gestrelius et al. 1997b; Petinaki et al. 1998). The pool of hydrophobic amelogenins, designated enamel matrix derivative (EMD), are expressed during cementogenesis and have been employed clinically for stimulating periodontal regeneration (Caton 1997). The enamel matrix derivative (EMD), dispersed in propileneglycol-alginate (PGA), is commercially available as Emdogain® (EMD) (Venezia et al. 2004; Zetterstrom et al. 1997) and is a biologic agent for stimulating periodontal regeneration (Sculean et al. 2002b).
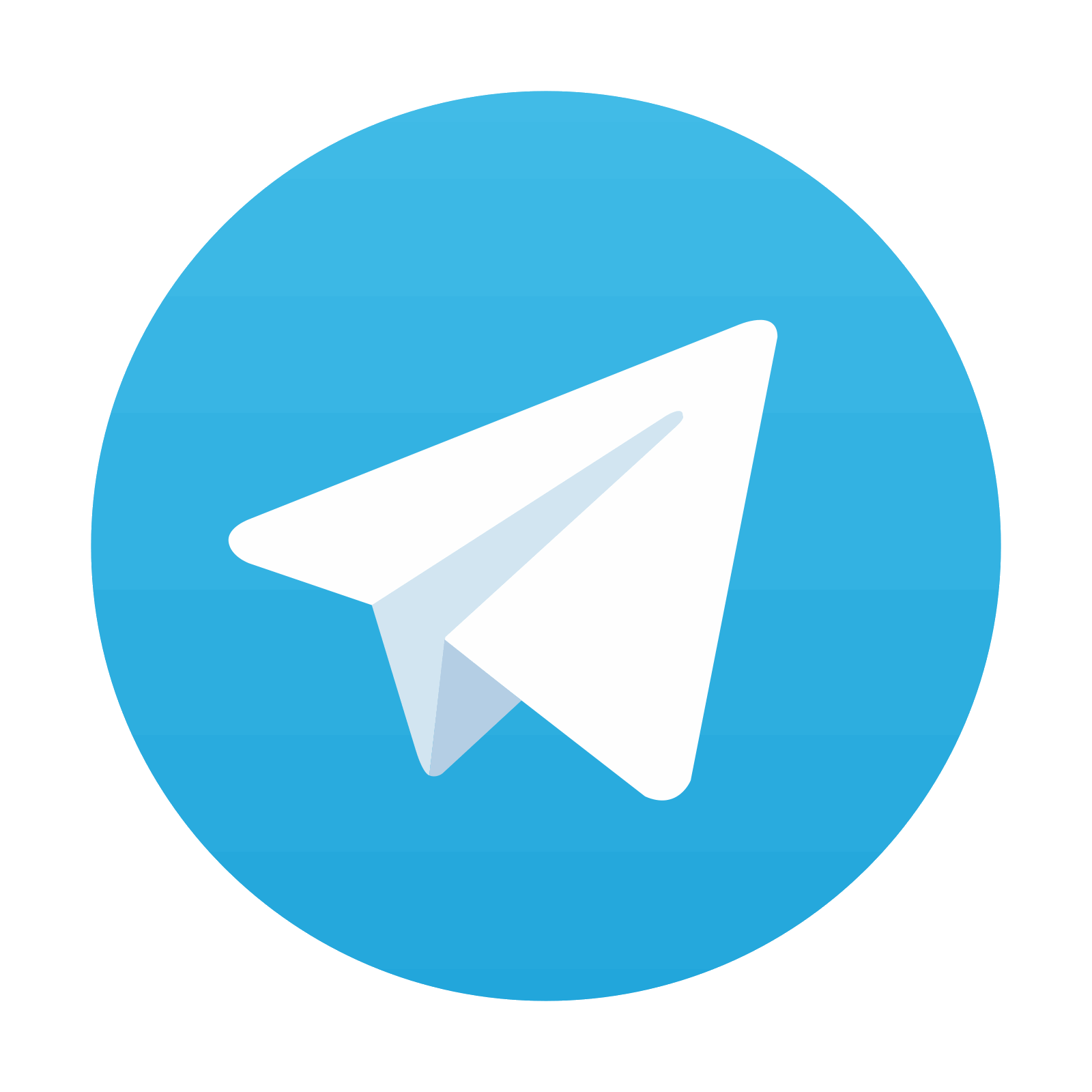
Stay updated, free dental videos. Join our Telegram channel

VIDEdental - Online dental courses
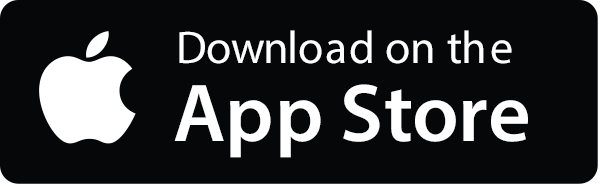
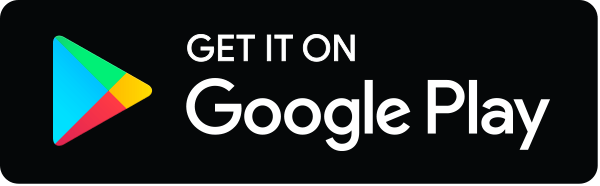