8
Considerations of Implant–abutment Connections for the Longevity of Dental Implants
Rafael Delgado‐Ruiz1, Fawad Javed2, and Georgios E. Romanos3
1 Department of Prosthodontics and Digital Technology, Stony Brook University, Stony Brook, NY, USA
2 Department of Orthodontics and Dentofacial Orthopedics, Eastman Institute for Oral Health, University of Rochester, Rochester, NY, USA
3 Department of Periodontics and Endodontics, Stony Brook University, Stony Brook, New York, USA
Introduction
There are two types of implant–abutment configurations: one‐piece and two‐piece. One‐piece design lacks interfaces as the implant body and abutment are continuous [1]. On the contrary, two‐piece configurations have an interface (implant abutment interface [IAC]) [1]. The IAC is defined as “the interlocking interfacial geometry between a dental implant and an abutment” and “the surface where the dental implant and prosthetic abutment connect”. These two components (implant and abutment) can be fastened via an abutment screw or by a friction system [2].
Mechanically, the resistance of the abutment against rotation is granted by an index system provided by different geometric configurations of the implant and abutment walls. This reduces rotational freedom to less than two degrees [3]; and resists dislodgement and micromovement at the IAC [4, 5]. In addition, tightening the abutment screw until preload is achieved stabilizes and retains both parts together thereby minimizing the risk of screw loosening [6, 7].
Biologically, appropriate sealing at the IAC interface reduces the migration of microorganisms inside the implant connection [8], and its apical‐coronal location determines the insertion levels for the stability of soft and hard peri‐implant tissues [9]. Clinically, the IAC also dictates the maintenance methods to support the longevity of osseointegrated implants [10].
The most coronal portion of the implant where the prosthetic abutment, restorations, and other prosthetic components connect with the implant is called the implant platform [11]. When correlated with the osseous crest, the implant platform serves as a reference to determine if an implant is subcrestal (apical to the crestal bone), crestal (at the same level with the crestal bone), or supracrestal located (coronal to the crestal bone). The edges of the implant platform coincide with the abutment edges in butt‐joint connections. However, it is not coincident in platform‐switching/shifting connections in which, edges of the implant abutment are mesial to the edges of the implant platform [11]. Significant geometrical differences exist in the different available IACs that may impact the frequency of mechanical and biological complications [12].
This chapter has three aims: first, to classify the different IACs designs; second, to compare their sealing capability against microorganisms; and third, to describe strategies for the maintenance of IACs.
Types of IAC
Characterization of IAC is based on the following features: (i) method of retention of the abutment to the implant, (ii) vertical positioning of the IAC in relation to the bone level and in relation to the implant platform, (iii) horizontal position of the IAC in relation to the implant platform edges, and (iv) geometric designs of the IAC (Figure 8.1).
Abutment Retention to the Implant Body
One‐piece Implants
One‐piece dental implants have been available for over five decades, and early examples were: blade implants, ceramic implants (synthetic sapphire and aluminum oxide), and titanium implants. The main characteristic of these designs is that the abutment is incorporated together within the same implant body [13]. Given that one‐piece implants are exposed to the oral cavity immediately after insertion, a second surgery is not required, and therefore they are also called “one‐stage” implants [14].

Figure 8.1 Classification of implant–abutment connections (IACs). Five groups are presented based on the method of retention, vertical position of the IAC in relation to the crestal bone, vertical position of the IAC in relation to the implant platform, horizontal position of the IAC in relation to the platform edges, and their designs. Multiple combinations and possibilities of these four groups are found in current dental implant systems.
The configuration of one‐piece implants (including the implant body and the abutment in a single piece) reduces the surgical trauma by eliminating a second surgery and simplifies the clinical procedure by eliminating the requirement for additional prosthetic components. In addition, the microgap is absent, which may reduce the bacteria counts [15]. Other advantages attributed to one‐piece implants are reduced crestal bone loss and inflammatory reactions, and biologic width dimensions similar to natural teeth [16]. Furthermore, surgical and clinical procedures for one‐piece dental implants results in less swelling, pain, time, and fewer appointments than procedures for two‐piece dental implants [17–19].
One‐piece dental implants are exposed to the oral cavity before they are osseointegrated; thus, they require primary stability (<30 Ncm) sufficient to withstand force vectors and to reduce micromovement‐related complications. Consequently, their use is recommended in areas with good bone quality and quantity [20].
When strict inclusion criteria are followed, one‐piece implants with immediate load have shown stable marginal bone and soft tissue levels and a high cumulative survival rate (98.8%) after two years in function [21]. The inclusion criteria used in the study by Finne et al. [21] described the following pre‐requisites for immediate loading on one‐piece implants: healthy subjects, good oral hygiene, stable occlusal relationships, no history of bruxism, sufficient bone volume, implants with at least 10 mm length, insertion torque between 35–45 Ncm, implant bed free of infection, and in the case of immediate implants, no extraction remnants. The authors also described the exclusion criteria for the use of one‐piece implants: lack of patient compliance, chronic bone diseases, bone tumors, previous irradiation, implant angles exceeding 10–15 degrees, and cantilevered prostheses [21].
Baer et al. [22] reported the results of a three‐years multicenter study using the same one‐piece implant system. In their protocol, implants were inserted and immediately temporized, and the final restorations were delivered after six months. One‐piece implants showed a cumulative survival rate of 98.3%, with minimal resorption of crestal bone, and preservation of the soft tissue levels [22].
Current one‐piece implants are also available in materials other than titanium, exemplified by one‐piece zirconia dental implants. Silva et al. [23] evaluated fatigue and failure modes of zirconia implants with a 30‐degree angle related to the vertical axis. The abutment portion of the implants was prepared to simulate the preparation for an anterior crown. The characteristics of the preparation included: a 2 mm occlusal reduction, a six‐degree wall angle, and a 0.5 mm chamfer reduction along the entire circumference. Forces over 600 Ncm were applied. The main failure mode for preparing an unprepared implant was a fracture, which occurred in the areas of exposed treads below the prepared abutment. Thus, demonstrating that a minimal preparation of the abutment portion of one‐piece zirconia implants did not increase the fracture incidence [23].
When the abutment portion of one‐piece implants is modified, the temperature can increase significantly at the level of the first threads. This was demonstrated in the study by Gabay et al. [24] which investigated the effects of the preparation of the coronal portion of one‐piece dental implants with and without irrigation on the temperature at the first thread of the implants. Their results showed temperatures over 47 °C reached between 5 and 11 seconds after the beginning of the abutment preparation in the group without irrigation. Furthermore, the temperature continued increasing after the drill stopped. Meanwhile, in the group with irrigation, the temperature elevation did not reach 47 °C. Thus, the authors demonstrated that the preparation of the abutment portion of a one‐piece dental increases the temperature and is essential to provide enough volume of irrigation to prevent fast temperature elevation at the level of the first thread [24].
In case of space limitations when narrow implants are recommended, it seems that the mechanical strength of one‐piece implants is superior to that of two‐piece implants; however, proper implant positioning is critical because the abutment orientation cannot be changed, and structural modifications of the abutment portion can result in a lack of retention of the implant crown or phase transformation in the case of ceramic implants [25].
It seems that the diameter of the one‐piece implant can influence the stability of the crestal bone [26]. Forty‐four narrow Implants (3 mm diameter) were inserted in healed ridges and in post‐extraction areas, and all the implants were immediately loaded with temporary crowns. Survival and radiographic bone levels were evaluated for one year. The survival rate after one year was 98%. The radiographic analysis of the bone le levels showed that 18% of implants presented <3 mm of bone loss, 20% of implants presented between 2 and 3 mm of bone loss, and 62% of implants presented >2 mm of bone loss after one year. Based on the excessive bone loss after one year, the authors recommended the cautious use of 3 mm diameter one‐piece implants [26]. There are also some limitations in using one‐piece implants, in case of implant malposition, the abutment requires modifications which results in titanium particle release, and the exposure to non‐axial loads can result in implant micromovement, lower success rates, and higher crestal bone loss [27–29].
Two‐piece Implants
Two‐piece implants are versatile and possess a different number of applications [30]. For example, in case of implant misalignment (17‐degrees up to 25‐degrees), custom or angled abutments can be used, if the implants lack primary stability, they can be submerged. In such cases, they require additional surgery after the bone healing to expose the implant platform and connect a healing abutment, also called “two‐stage” implants [31].
Two‐piece titanium implants (100%) have survival rates comparable to those of one‐piece implants (100%) after five years [32, 33]; however, it seems that they present more technical complications (screw loosening and porcelain fractures) [32, 33].
The transmucosal portion of the prosthetic abutments of two‐piece dental implants can be customized to accommodate soft tissue thickness and biologic width [34], the abutment angle can be modified to correct up to 25‐degree implant misalignment [35–37]. The implant can be submerged in augmented areas, lack of stability, or if a removable denture is being inserted and risk of excessive uncontrolled forces is present [38–40].
Some differences between two‐piece and one‐piece dental implants include: the gingival margin being more coronal in one‐piece implants and the biologic width dimensions being more similar to natural teeth in one‐piece implants [16]. Furthermore, the presence of an interface in two‐piece dental implants and microgap, allows potential micromovement, bacteria colonization, and screw loosening [41].
The prosthetic abutment can be retained in two‐piece dental implants by an abutment screw or a friction system (screw‐less). In screw‐retained abutments, the retention screw is tightened to produce an elastic deformation (flattening of the microrough edges of the screw threads and elongation of the screw) achieving enough vertical compressive force between the implant and the abutment (preload) to reduce screw‐loosening [42, 43]. It has been demonstrated that 90% of the tightening force is spent as frictional force and 10% of the force is converted to preload [44].
In implant abutments retained by friction systems, a morse taper connection produces high tension between the abutment and the implant and a horizontal cold‐welding effect. Taper angles around 1.5° and 4.0° degrees ensure an excellent coupling between the mating surfaces of the abutment and the implant connection [45, 46]. The friction‐retained abutment systems seem to function comparably to screw‐retained abutment systems [47].
Vertical Implant Position of the IAC in Relation to the Bone Level
The implant platform can be placed at three alternative vertical positions at the moment of implant insertion: supracrestal, crestal, or subcrestal. In supracrestal positions, the implant platform is elevated over the crestal bone; therefore, the IAC is also located over the bone crest. In crestal positions, the implant platform edges, and the IAC are leveled with the adjacent bone, and in subcrestal positions, the implant platform and the IAC are below the crestal bone level [2].
The consequences of different implant insertion depths are variable. For example, it was found that the placement of the IAC at or below the alveolar crest did not influence the peri‐implant crestal bone levels after five years in human studies [48]. However, posterior studies that used implants with platform‐switching (abutment diameter narrower than implant platform diameter) demonstrated that subcrestal placement presented less crestal bone loss than platform‐switched implants placed at the crestal level [49]. Increased number of neutrophils and moderate bone resorption occur when the IAC is located at the level of the bone crest and If the IAC is superior to the crestal bone (in platform‐switching or one‐piece implants), minimal inflammatory infiltrates and lower bone resorption can be expected [1].
Contradictory results were presented in recent systematic reviews and meta‐analyses that evaluated the bone loss of implants placed at subcrestal or crestal level [50, 51]. In the study by Palacios‐Garzón et al. [50] sixteen studies were included. Ten studies did not show differences in bone loss between crestal or subcrestal placement, three studies found more crestal bone loss in subcrestal placement, and the other three found more bone loss in crestal placement.
In the most recent systematic review and meta‐analysis by Cruz et al. [51] the marginal bone loss, implant survival, and soft tissue parameters around implants placed crestal or subcrestal were evaluated. The authors included ten studies that fulfilled the inclusion criteria. The meta‐analysis showed that implant depths did not influence implant survival or other peri‐implant parameters (marginal bone loss, probing depths, or keratinized tissue).
Rationale for Crestal or Subcrestal Implant Placement
Implant placement is followed by a remodeling process that results in a marginal bone loss between 0.25 and 0.65 mm during the first year [52, 53]. In both cases (crestal and subcrestal placement), the remodeling is comparable, but in subcrestal placement lower risk of exposed threads can be expected [54]. This occurs because re‐establishing the biological width around implants requires a minimum distance of 2 mm between the implant platform and the gingival surface. Therefore, by adapting the implant insertion level to the surface of the soft tissues bone remodeling can be controlled [55, 56].
In the case of soft tissues with thin phenotypes, the establishment of the biologic width can occur by causing bone resorption [57]; meanwhile, in the case of thick gingival phenotypes, the marginal bone resorption is reduced [58, 59]. Finally, the marginal bone level is affected by the implant design + implant insertion depth combinations [53]. For example, tissue‐level implants (with an incorporated transmucosal portion) preserve the bone if inserted at crestal level; meanwhile, bone‐level implants preserve the bone when placed subcrestally [53].
All the previous can be applied to implants inserted in the healed bone. However, when implants are inserted in post‐extraction sockets, more bone remodeling is expected, specially at the buccal bone area, where resorption up to 2.2 mm has been reported after tooth extraction [60, 61]. Therefore, in post‐extraction sockets, the bone resorption can be compensated by completing a deeper implant insertion from 2.5 to 3.0 mm below the crestal bone [62]. The insertion depth range in the case of post‐extraction implants with platform‐switching is smaller than implants without platform‐switching, and 1.08 mm of subcrestal insertion is enough in platform‐switched implants to avoid exposure of the implant neck [63].
Vertical Position of the IAC in Relation to the Implant Platform
Two main configurations for IACs have been described in two‐piece dental implants: external or internal. In external IACs, the connection occurs over the body of the implant at the implant platform, and the center of rotation of the abutment is high resulting in abutment instability. Meanwhile, in internal IACs, the connection occurs within the implant and below the implant platform, and the center of rotation of the abutment is low, resulting in higher abutment stability [64].
The external connection was intended to serve for the insertion of the implant and to stabilize the implant supra structure [65]. However, the height of the geometrical feature of external connections (mainly hexagonal) was inefficient in reducing micromovement against non‐axial loads of elevated magnitude. Thus, conducting to screw loosening, and fatigue and fracture of prosthetic screws [66].
Internal connections were developed to limit the drawbacks of external connections (screw loosening, micromovement, and fracture). Internal connections possess different depths, geometries, and mating properties (between the internal walls of the implant and the abutment connection) that seem to improve the transfer of forces and reduce abutment micromovement and screw loosening [64, 67].
Other claimed benefits of internal connections over external connections have been reported in in vitro and in vivo studies, including better stress distribution along the implant body when non‐axial loads are applied, better maintenance of the screw preload, lower Interleukin 1 (IL‐1) and tumoral necrosis factor‐α (TNFα) around the implants [68], and lower screw loosening and mechanical complications observed in clinical studies with at least three years of follow‐up [69].
Finally, it has been demonstrated that wider microgaps appear in external connections when lateral forces are applied, favoring bacterial microleakage [70], and better esthetic outcomes are observed around implants with internal connections placed in the anterior region compared to those with external connections [71].
Horizontal Position of the IAC in Relation to the Platform Edges
Two horizontal positions of the IAC in relation to the implant platform edges have been described: butt‐joint and platform‐switched positions. The main difference is that the implant and the abutment match in butt‐joint positions and not in platform‐switched positions [72]. In butt‐joint IACs, the edges of the abutment and the implant platform are coincident without overlapping. Therefore, the diameter of the platform and the basal diameter of the prosthetic abutment are the same. Meanwhile, in platform‐switched, the basal diameter of the implant abutment is narrower than the implant platform [73].
In platform‐switching, the inward displacement of the abutment walls toward the central axis of the implant creates a step on the implant platform [74], (a horizontal space) that allows for the horizontal establishment of the biologic width and bone ingrowth [75]. Once the abutment contour is moved away from the edges of the implant platform, the implant–abutment microgap and the associated inflammatory infiltrate are also distanced from the marginal bone thus, reducing bone resorption [76].
Given that implant diameters vary in range from narrow to wide implants, a platform‐switched abutment may possess different levels of mismatch. Canullo et al. [77] studied the effects of an abutment with 3.8 mm diameter inserted on implants with different diameters (3.8, 4.3, 4.8, and 5.5 mm). Thus, total mismatches of 0, 0.25, 0.5, and 0.85 mm were evaluated. The authors observed that after three years of follow‐up, larger mismatch resulted in lower marginal bone resorption [77]. This was confirmed by histological studies completed in minipigs with platform‐switching of 0.25 mm [78], and in radiographic studies with platform‐switching up to 0.85 mm (in which implants with platforms with 5.8 mm diameter received 4.1 mm abutments) that demonstrated decreased marginal bone resorption compared with non‐platform‐switched abutments [79, 80].
Another aspect related to the marginal bone preservation observed in platform‐switching is the soft tissue thickness. When sufficient apical‐coronal and buccal soft tissue thickness are present, the marginal bone is preserved in both platform‐switched and non‐platform‐switched implants. However, when the tissue thickness is reduced, platform‐switching is efficient in preserving the marginal bone level [81].
Vandeweghe et al. [81] completed a randomized controlled trial in patients who received implants with wide platforms (7 mm) and connections centered in the implant axis or with an offset (two platform‐switching sizes 0.225 or 1.225 mm). After 3, 6, and 12 months radiographs were obtained to evaluate the marginal bone levels at the mesial and distal sides. Their results showed that the marginal bone loss decreased by 30% in platform‐switched implants when the soft tissue thickness was sufficient [81].
The biomechanics of implants with platform‐switched abutments have also been considered responsible for reduced bone loss [82–84]. When oblique forces are applied on platform‐switched abutments, the functional stresses are re‐directed to the center of the implant, thus transferring the strains to the cancellous bone, and protecting the cortical bone [82–84].
Moreover, under function and simulated accelerated corrosion, implants with platform‐switching released fewer metal ions than implants with butt joint connections. Implants with butt joint connection presented active corrosion at the outer borders of the contacting surfaces. Meanwhile, platform‐switched connections released minimal amounts of titanium ions which may explain the reduced bone loss observed around platform‐switched IACs [85, 86].
A recent systematic review and meta‐analysis by Mishra et al. [87] evaluated randomized controlled trials with at least three years of follow‐up comparing platform matching with platform‐switching. The results showed that platform‐switching limited the marginal bone loss better when the mismatch between the implant and the abutment was greater, the maxilla reacted better than the mandible to platform‐switching, and better bone preservation with platform‐switched implants with splinted prostheses inserted at bone level [87].
Geometric Design of the IAC Index
Implant manufacturers are working on improving the IAC index designs to reduce abutment rotation, improve load distribution, reduce bacteria filtration, and minimize screw loosening to limit mechanical and biological complications [88]. Common IAC index designs are based on angles, channels, morse‐taper (conical), and combinations of different geometries [89]. Each IAC index design possesses certain variability. For example, the angled index connections can be hexagonal, octagonal, and dodecagonal (this means 6, 8, or 12 angles); channel index type connections can have 3, 4, 6, 7, or 8 channels (with straight or round contours) with variable dimensions, and morse tapered connections are available with 4‐degree to 11‐degree taper angles and different depths and can have or not an index.
Further available IACs include a combination of geometries like morse taper + hexagon, or conical + hexagon, and unique geometries like spline type, and triangle conical. The following subsections review the characteristics of each group.
Angled Indexed Connections
Angled connections are available with 6, 8, or 12 angles, and all possess different rationales that support their use. In the following subsection, details about each type of connection are provided.
Connections with Six Angles (Hexagonal)
The oldest of the angled IACs is the hexagonal connection initially described by Branemark in 1969 [90]. This design simplified the implant insertion, provided anti‐rotational support for the restoration on single implants [70], and allowed for one‐ or two‐stages implant surgery [70,88–90].

Figure 8.2 (a) Internal hexagon. The dark outline is the contour of the implant. The silver ring is the implant platform. (b) Illustrates in red color the hexagonal indexed area, and in green, each one of the vertex of the hexagon.
The hexagonal connection can be internal or external, and some implant systems simultaneously possess internal and external hexagons [91]. Hexagonal connections present some limitations; for example, the short height of the external hexagon under non‐axial forces can allow micro movement and frequent screw loosening in single implant restorations, and the prosthetic screw is the only component retaining the butment [64, 92]. Also, when non‐axial loads are applied on external hexagonal connections, the dimensions of the microgap increase, conducting in increased counts of microorganisms inside the IAC [93]. In addition, as the microgap increases the range of micromotion also increases. This, in turn, increases stresses that are transferred to the vertices of the hexagon initiating a cycle of wear, more micromotion, and potential fractures [94] (Figures 8.2 and 8.3).
Connections with Eight Angles (Octagonal)
Octagonal connections were designed to improve the also‐called “morse‐taper” connection. The morse‐taper connection consists of a cone – fitting within – a cone, and the precise matting between both conical surfaces achieves the stability of this connection [95]. The taper angle variates between 4 and 11%, and total convergence angles can be up to 16 degrees [96]. However, there were some difficulties in transferring positional information between the clinic and the laboratory because the cones are symmetric and do not possess an anti‐rotational element [97]. Including an index (hexagon, octagon, and others) below the cone, provided reproducibility between the dental laboratory and the clinical procedures and reduced micromotion [94, 98].
Setbacks of conical connections with octagonal index are that a vertical sliding movement occurs during the insertion of the abutment to achieve friction fit. Some octagonal connections do not have a stop element, and a settlement phenomenon (the abutment is slightly intruded into the implant) can occur when high vertical occlusal loads are applied, resulting in changes in the occlusal height of the restoration [99].
Another potential issue of conical implant–abutment conical + octagonal index is that after simulated repeated loading (vertical load of 250 N for 100,000 cycles) representing the stresses received for one month, the abutment removal torque decreases from 24 ± 0.43 to 17.66 ± 0.59 Ncm, and the vertical displacement can reach up between 5.0 ± 1.8 and 8.8 ± 1.10 μm [100] (Figure 8.4).
Connections with 12 Angles (Dodecagonal)
Connections with 12 angles were designed to increase the restorative options by adding more positional possibilities to the prosthetic abutment. The implants with a dodecagonal index also possess a hexagon that engages the implant driver. Thus, the dodecagonal index is protected from deformation in high insertion torques [101, 102]. The dodecagonal index enables the surgeon to place the flat surfaces of the implant connection in any position during the surgery. The prosthetic abutment can be oriented in 12 different positions. This allows increased rotational flexibility [101]. In mechanical studies with finite element analysis, it was determined that oblique forces applied to three different IAC designs (external hexagon, internal dodecagon, and internal conical tapered hexagon with 45° angle), were more stable distributed and less displacement observed in dodecagonal internal connections [102] (Figure 8.5).

Figure 8.3 Internal hexagon with internal bevel. The picture is showing an implant system that possesses a platform beveled internally in a slight tapered angle. The external silver‐light ring is the implant platform, the dark ring is the superior part of the bevel, the third silver‐ring is the lower part of the bevel, the hexagonal index area is highlighted in red color. The vertex of the angles is indicated with blue numbers. Digital microscope image at 50X.

Figure 8.4 Internal octagon. This system presents a platform narrower than the implant diameter. The external dark ring is the implant body, the internal silver ring is the platform, and inner to the platform is the octagonal index area highlighted in red color. The vertex of the angles is indicated with green numbers. Digital microscope image at 50X.
Channel Indexed Connections
The channeled connections are also called grooved indexed connections, which are present in tube‐in‐tube and conical connections. The main characteristic is that in the tube‐in‐tube connection, the channel (or groove) is present at the coronal level of the main tube; meanwhile, in conical connections, the tapered wall ends in a short cylinder that contains the channels (thus, the channels are more apical) [103–108].

Figure 8.5 Implant with dodecagonal index. This figure illustrates an implant with a flat implant platform in yellow color. Internally, in red color a hexagonal area is highlighted. The center, a dodecagonal index area is indicted in blue color. The angles of the hexagon are indicated in red, and the angles of the dodecagon in black color. Digital microscope image at 50X.
Channeled connections can have three, four, six, or eight symmetric channels (i.e. trilobe®, conelog®, cross fit®), which allow for three, four, six, or eight abutment positions. In addition, there are also channeled connections with asymmetric channels (indexing dots) that allow only one abutment position [103–108].
The channels can be rounded or squared form (like boxes) and have different widths and depth sizes. The channeled index design possesses certain advantages, including good reproducibility of implant/abutment position, excellent rotational stability, and good distribution of loads [103–105].
One disadvantage of the channel in some tube‐in‐tube connections is that their location at the coronal area and the channel size can produce a thinning of the implant wall that can result in fractures in narrow implants [94, 95]. However, in wider implants, the tube‐in‐tube channeled connection can increase the fracture strength and the stability of the restoration, and the apical location of the channels in conical connections results in thicker implant walls and a lower risk of fracture [94, 106, 107]. Overall, the presence of channels or grooves significantly reduces the vertical, horizontal, and rotational movement of the prosthetic abutment [108] (Figures 8.6–8.10).
Morse Tapered Connections
Morse tapered connections are also called conical connections. The morse taper design possesses conical walls with internal angles of different degrees. For example, implants with morse tapered internal walls with 8‐ to 11‐degrees taper angles, showed increased resistance to screw loosening, and increased mechanical strength [109–111]. Conical connections with a 4‐degrees tapering angle increase the frictional contacts between the implant and the abutment, providing enhanced anti‐rotation and improved sealing against fluids and bacteria leakage. This precise fit is more efficient in transferring horizontal forces from the abutment to the implant and reduces the stresses received by the abutment screw [112, 113].
The fracture resistance of abutments with morse tapered connection (conical) compared with internal hexagonal connection was tested under oblique compressive forces. Morse tapered connections with 3.5 connection depth, and 11.5‐taper angle showed higher deformation torque values (90 ± 6.72 Kgf) than internal hexagonal connections (83.73 ± 4.94 Kgf) [114].
The performance of morse tapered designs was evaluated in a systematic review that compiled in vitro and in vivo studies. In vitro studies demonstrated that morse tapered conical connections presents a superior bacterial seal, increased resistance to abutment displacement, minimal microgap, higher resistance to torque loss and fatigue, and less transmission of stresses to the abutment screw. In vivo studies showed reduced crestal bone loss, success, and survival comparable to other connection systems [115]. In addition, the conical abutment that engages in the morse tapered connections presents a smaller diameter than the implant platform, which provides a platform‐switched configuration. Therefore, morse tapered designs allow increased space for establishing the biologic width and increased connective tissue thickness surrounding the abutment [116].

Figure 8.6 Implant with six channels index. This figure illustrates an implant with flat platform, internally, a small cone that ends in six symmetric boxes separated by the channels indicated with green numbers. In red color, the area between boxes that contain the channels is highlighted. Digital microscope image at 50X.

Figure 8.7 Implant with seven channels index. This figure illustrates an implant with flat platform, internally, a cone that ends in seven asymmetric boxes separated by the seven channels indicated with green numbers. In red color, the area between boxes that contain the channels is highlighted. Digital microscope image at 50X.
Morse taper conical abutments were subject to cyclic axial loads in the presence of biofilm, and the removal torque values were evaluated against the baseline. The results showed that the removal torque increased significantly after repeated load from 27.2 ± 7.5 Ncm to maximum values of 160.6 ± 15.8 Ncm after 100,000 cycles and up to 154 ± 14 Ncm after 500,000 cycles [117]. This confirms the increased removal and the active settlement under load experienced by morse taper connections without a stop design.

Figure 8.8 Implant with an index with eight rounded channels. This implant shows an outer dark gray ring (implant body), a silver ring (a flat implant platform), and eight symmetric rounded channels in red. Each round channel is indicated with green numbers. Digital microscope image at 50X.

Figure 8.9 Zirconia implant with a four channels index. These photos are showing a zirconia implant with a flat implant platform. Internally in red color, four channels are highlighted in red color. Digital microscope image at 50X.
Finally, a recent prospective study with follow‐up from one to nine years evaluated morse taper connections with and without positional index. The study showed that indexed abutments suffered more fractures than non‐indexed abutments in molar positions. In addition, the survival of non‐indexed morse cone abutments was higher (99%) than morse cone indexed abutments (95%) [118] (Figures 8.11–8.13).

Figure 8.10 Implant with a tube‐ in tube connection with three channels. (a) The inner walls of a tube‐in‐tube connection are almost parallel however, this image example shows 1‐degree taper angle to facilitate the insertion of the abutment. (b) Coronal view of a 3.5 mm diameter implant ant the three rounded channels. (c) 3D‐reconstruction from the characteristic inner cavity of the implant with three channels. Digital microscope image at 50X.

Figure 8.11 Implant with morse cone tapered connection. This implant system shows the platform (flat and wide) in gray color. Inside, a blue area indicates the internal cone. In yellow a six channels index, and in red is indicated the basal space for the prosthetic screw. Microscope image at 50X.

Figure 8.12 Section of the IAC of an implant with morse cone tapered connection. The cone is located at the most coronal portion of the IAC. The index area is inferior to the cone, and in red the basal area is the space for the apical portion of the prosthetic screw. Obtained by vertical scanning with the digital microscope and lenses of 100X.

Figure 8.13 Implant with morse tapered connection. The dark color is the implant body beveled internally toward a silver ring (implant platform). In yellow, the morse tapered area, and inside in red, a hexagonal index. The numbers in green color are indicating the angles of the hexagon. Microscope image at 50X.
IAC Design and Sealing Capability Against Bacteria Colonization
Microorganisms can penetrate and colonize the implant–abutment interface demonstrated by the presence of colonies of microorganisms at different areas inside the implant connection, in screw‐retained and in cement‐retained restorations [119, 120] Given that the dimension of the microgap differs between IAC designs, the percentage of bacteria that can penetrate inside the implant connection variates. For example, higher counts of microorganisms are measured for external connections compared to internal connections. Furthermore, within internal connection designs, the conical connections have shown less bacterial counts compared to non‐conical connections [122, 123]. The morse‐taper connection is the most resistant to bacteria penetration although, smaller molecules (endotoxins) can penetrate all types of connections including morse‐taper connections [124, 125]. The microbial contamination of the IAC can occur by multiple causes (handling, lack of fit between components, design of the IAC, and translocation of microorganisms). In this section, the potential mechanisms that can produce microbial contamination inside the IAC, as well as the effect of the IAC design in the bacteria colonization are summarized.
Mechanisms for Microbial Contamination of the IAC
Microbial contamination of the IAC can be induced by implant insertion [126–132], before the restoration is delivered, and during function [129]. During the fabrication of the implant restoration, microbial contamination of the abutments can occur, and contaminated abutments can transfer this contamination to the IAC during the insertion of the implant restorations [130].
Penarrocha et al. [127] evaluated the microbial colonization produced by the insertion of dental implants after five years post‐loading. Two types of implants were compared pre‐mounted and mount‐less dental implants. The difference between both systems is that pre‐mounted implants come with an insert that is released after the implant insertion, and mount‐less implants come without an insert and are transferred to the implant bed using universal implant drivers.
After five years, samples were obtained from the implant connection, and higher counts of bacteria were observed in the implant inserted with the universal drivers compared to implants inserted with pre‐mounts. Given that the implant driver transfers rotational forces to the implant index, and the fit of the pre‐mounted insert surpasses the fit of the universal implant driver, the authors explained that the deformation of the connection during insertion induced by the driver was higher than the deformation of the connection induced by the pre‐mount and recommend the use of pre‐mounted dental implants and reduce the insertion torques [127].
In addition, De barros Lucena et al. [130] obtained samples of the implant connection at the second stage of surgery. Careful isolation of the implant area was completed and after the removal of the cover screws, paper points were used to obtain samples of the implant’s intaglio. The samples were evaluated by checkerboard DNA hybridization. Their results detected 22 different species of bacteria in 62.5% of the subjects, and a higher percentage of bacteria was found at the mandible (77.42%) compared to the maxilla (36.17%) [130] (Figure 8.14).
After exposure to the oral environment, healing abutments will suffer from surface contamination by saliva, food debris, microbial and epithelial cells, and nicotine (in smokers) [132, 133]. The removal of all contaminants from healing abutments by conventional cleaning, or their complete sterilization has not been achieved because traces of proteins and amino acids are still detectable in microbiological evaluations of healing abutments after different cleaning and sterilization regimes [134].
Cover screws removed during the second stage surgery also showed contamination, including tissue debris, bacteria, and corrosion products not observed in new cover screws [135]. The histological analysis of the soft tissues located over cover screws also showed chronic inflammatory infiltrate. This could mean that although clinically the mucosa appears healed, a communication tract persisted between the cover screw and the oral environment, or the cover screws were contaminated during insertion [136]. Therefore, healing abutments and cover screws can be other sources of contamination of the IAC (Figure 8.15).
Once microorganisms colonize the inner aspect of the implant, the IAC becomes the perfect reservoir where bacteria are protected, larger colonies can grow and where a transition to a microflora that is mainly anaerobic and more pathogenic, is common [137]. The dynamics of fluid flow within implant connections still need to be understood. Factors that include saliva viscosity, composition and volume of saliva, and geometrical constraints can affect the microorganism’s attachment and accumulation at the implant connection [138] (Figure 8.16).
Also, fluid flow can affect the transport and physiology of the microbial residents by inducing phenotypic changes [139]. In small spaces, fluid tension produces an accumulation of motile bacteria close to the sidewalls and decreases bacterial concentration in the central regions of channeled spaces [140–143
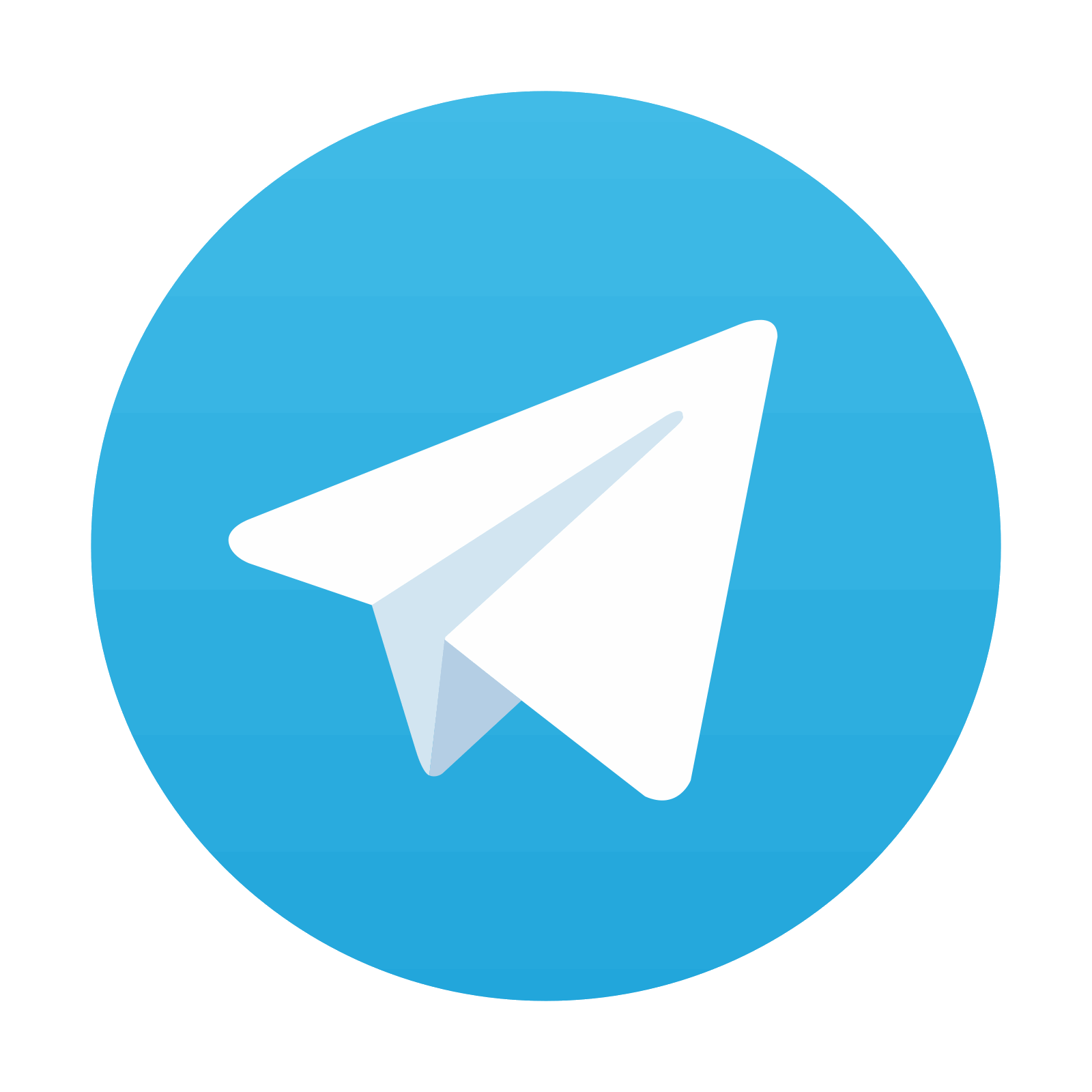
Stay updated, free dental videos. Join our Telegram channel

VIDEdental - Online dental courses
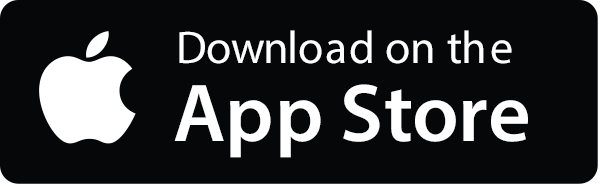
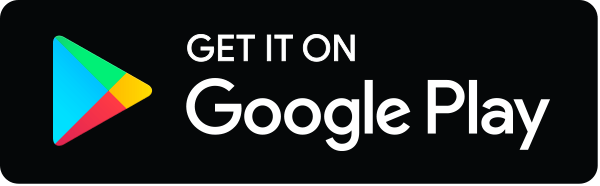