Abstract
Objective
To evaluate the ability of thio-urethane oligomers to improve the properties of restorative composite resins.
Methods
Oligomers were synthesized by combining 1,6-hexanediol-diissocyante (aliphatic) with pentaerythritol tetra-3-mercaptopropionate (PETMP) or 1,3-bis(1-isocyanato-1-methylethyl)benzene (aromatic) with trimethylol-tris-3-mercaptopropionate (TMP), at 1:2 isocyanate:thiol, leaving pendant thiols. Oligomers were added at 0–20 wt% to BisGMA-TEGDMA (70–30 wt%). Silanated inorganic fillers were added (70 wt%). Materials were photoactivated at 800 mW/cm 2 filtered to 320–500 nm. Near-IR was used to follow degree of methacrylate conversion (DC). Mechanical properties were evaluated in three-point bending with 2 mm × 2 mm × 25 mm bars for flexural strength/modulus and toughness (FS/ E , and T ) according to ISO 4049, and 2 mm × 5 mm × 25 mm notched specimens for fracture toughness ( K IC ). Polymerization stress (PS) was measured on the Bioman. Results were analyzed with ANOVA/Tukey’s test ( α = 5%).
Results
Significant increase in DC was observed in thio-urethane-containing materials especially for the group with 20 wt% of aliphatic version. Materials composed by oligomers also promoted higher FS, E , and K IC in comparison to controls irrespective of thio-urethane type. A significant increase in toughness was detected by ANOVA, but not distinguished in the groups. The PS was significantly reduced by the presence of thio-urethane for almost all groups.
Conclusions
The use of thio-urethane oligomer to compose methacrylate-based restorative composite promote increase in DC, FS, E and K IC while significant reduces PS.
Significance
A simple additive was shown to reduce stress while increasing convrersion and mechanical properties, mainly fracture toughness. This has he potential of increasing the service life of dental composites, without changing current operatory procedures.
1
Introduction
Resin-based composites are widely used in Restorative Dentistry due to their highly esthetic appearance and the possibility of performing minimally invasive cavity preparation. Although these characteristics are advantageous, the main drawback with these materials is that they last on average less than 10 years in the mouth . The most common causes of composite restoration failure are secondary caries and fractures . It has been suggested that the gaps formed due to stress generation at the bonded interface may facilitate caries re-occurrence.
The stress development is caused by a multifactorial process that includes the degree of conversion and the consequent development of shrinkage and modulus during curing, as previously reported . Volumetric shrinkage occurs simultaneously with the increase in the elastic modulus, as the density of cross-linked bonds among the polymeric chains increases . Also, the polymerization reaction rate, the material’s composition and the surrounding conditions around the restoration (bonding integrity, C-factor, and deformation of the adjacent structures) are important factors regulating the development and transmission of stress to the bonded interface and to dental structures .
A series of reports pointed out that the behavior of composite resins in bending is an important predictor of the clinical performance . Ferracane has shown a direct relationship between mechanical properties such as fracture toughness, flexural strength, elastic modulus and toughness and clinical outcomes, suggesting that the improvement of these mechanical properties may contribute to better clinical performance and longevity of the restorations. Most of the advances in the formulations that have made it possible to improve mechanical properties of current composite materials stem from changes in the filler (inorganic) portion of the composite . One of the weak links remains the hydrolysis-prone methacrylate organic matrix , which has not changed significantly since the introduction of BisGMA on the market more than six decades ago.
Based on the clear shortcomings of current materials, there is a need for formulations with the ability to develop lower stress to show less degradation and improved mechanical properties, which would indeed decrease the variability of the outcomes . Earlier studies have demonstrated the ability of materials based on thiol-enes or thiol-methacrylate to increase conversion and mechanical properties such as fracture toughness, at the same time reducing stress and water sorption/solubility . This is possible due to delayed gelation/vitrification of the thiol-modified networks, and has been demonstrated for several small molecule and oligomeric thiol species . Very recently, thio-urethane oligomers were shown to promote more homogeneous network formation compared to simple urethane counterparts leading to increase in degree of conversion and in mechanical properties, especially toughness and fracture toughness . In the case of thio-urethane oligomeric additives, the higher molecular weight leads to lower volumetric shrinkage and absence of odor concerns . Still, the chain-transfer reaction of the pendant thiols to the surrounding methacrylate matrix results in delayed gelation and vitrification, promoting reduction in polymerization stress .
The objectives of this study were to formulate composite materials modified with thio-urethane additives previously synthesized in our laboratory and to assess the influence of such additives on degree of conversion and reaction kinetics, bulk mechanical properties, polymerization shrinkage and stress. The hypotheses of this study were that, depending on the type and concentration of the thio-urethane, the addition of oligomers will lead to (1) increased degree of conversion; (2) increased mechanical properties; and (3) reduced polymerization stress of methacrylate-based composites.
2
Materials and methods
2.1
Experimental materials composition
The composites formulated for the study were composed of Bisphenol A diglycidyl methacrylate (Bis-GMA; Esstech, Essington, PA, USA) and tri-ethylene glycol dimethacrylate (TEGDMA; Esstech) in a 70:30 mass ratio. Photoinitiators were added to the matrix as follows: 0.1 wt% of 2,2-Dimethoxy-2-phenylacetophenone (DMPA – Sigma–Aldrich, St. Louis, MO, USA) and 0.3 wt% inhibitor (BHT – 2,6-di-tert-butyl-4-methylphenol; Sigma–Aldrich, St. Louis, MO, USA).
Oligomers were synthesized in solution by combining 1,6-hexanediol-diissocyante (aliphatic) with pentaerythritol tetra-3-mercaptopropionate (PETMP) or 1,3-bis(1-isocyanato-1-methylethyl)benzene (aromatic) with trimethylol-tris-3-mercaptopropionate (TMP), at 1:2 isocyanate:thiol molar ratio, leaving pendant thiols. Oligomers were purified by precipitation in hexanes and rotaevaporation, then characterized by 1 H NMR and mid-IR spectroscopy. The thiol group (SH) concentration for each oligomer was determined using a titration method with Ellman’s reagent well established in the literature . Thio-urethane oligomers were added to organic matrix in proportions of 0–20 wt%; no thio-urethane group served as control.
Filler was introduced at 70 wt% (7% OX-50 – 0.04 mm; 93% silica 0.7 μm, density 3.0 g/ml, refractive index 1.553 – V117 4107, Esstech), with the aid of a mechanical mixer (DAC 150 Speed mixer, Flacktek, Landrum, SC, USA) for 5 min at 2400 rpm. All procedures were carried out under safe yellow light.
2.2
Degree of conversion
Degree of conversion based on the methacrylate CH 2 absorption at 6165 cm −1 was calculated based on near–infrared (NIR) spectroscopy at 2 scans per spectrum with 4 cm −1 resolution, which provides a greater than 2 Hz data acquisition rate. Samples ( n = 3) were irradiated during the course of the analysis (5 min) to account for thermal effects during the kinetics experiment, at an incident irradiance of 800 mW/cm 2 filtered to 320–500 nm with a 2 cm distance to specimen. This light source was used for convenience and stability of the irradiance through long exposure times. Specimens were 10 mm in diameter and 0.8 mm thick laminated between two glass slides.
2.3
Flexural strength, elastic modulus and toughness
Flexural strength of the samples was measured according to the 3-point bending method carried out with a universal test machine (Q-test, MTS, Eden Prairie, WI) at a cross-head speed of 0.5 mm min −1 . The bar specimens ( n = 10) were prepared in dimensions of 2 mm × 2 mm × 25 mm according to ISO 4049 . The specimens were fabricated between glass slides and photopolymerized with 300 s exposures as mentioned above. Specimens were stored for 1 week in dark containers at room temperature. The flexural strength (FS) in MPa was then calculated as:
FS ( σ ) = 3 F l 2 b h 2
where F stands for load at fracture ( N ), l is the span length (20 mm), and b and h are the width and thickness of the specimens in mm, respectively.
The elastic modulus was determined from the slope of the initial linear part of stress–strain curve.
E = F l 3 4 b h 3 d
F = the load at some point on the linear region of the stress–strain curve; d = the slack compensated deflection at load F ; l , b , and h are as defined above.
Toughness was calculated in MPa from the integration of the stress × strain curve using software (Origin 9.1, OriginLab Corporation, Northampton, MA, USA).
2.4
Polymerization stress
Polymerization stress development was followed in real-time using the Bioman, described previously . This system consists of a cantilever load cell whose extremity is fitted to a rigid integral clamp on its free end. The clamp holds a 10 mm diameter and 22 mm tall steel rod vertically and perpendicular to the load cell axis. A 5-mm diameter, 1-mm tall steel rod was fixed at the center of the lower face of the standard rod with a cyanoacrylate adhesive to produce a rod substrate with a reduced surface area to be consistent with a C-factor of 3. The surface of the rod was treated with a thin layer of metal primer (Z-prime plus, Bisco, Schaumburg, IL). The opposite surface was a rigid fused silica glass plate of 3 mm thickness, treated with a thin layer of silane ceramic primer (3M ESPE, St. Paul, MN, USA). The composite was then inserted into the 0.5-mm gap between the upper rod and the lower glass slide and shaped into a cylinder. The specimens were photoactivated through the glass during 60 s at an incident irradiance of 800 mW/cm 2 (UV curing light) and the stress followed for 500 s. The load signal from the cantilever cell was amplified and acquired by a computer.
2.5
Fracture toughness
The method utilized to determine fracture toughness ( K IC ) was based on the evaluation of pre-cracked specimens under fatigue in linear-elastic, plane-strain conditions . Single-edge notch beam (SENB) specimens were fabricated according to ASTM Standard E399-90 in a 5 mm × 2 mm × 25 mm split steel mold with a razor blade providing a 2.5 mm notch in the middle of the specimens. Specimens were cured with an UV light during 300 s at 800 mW/cm 2 irradiance filtered to 320–500 nm. The bending fracture test was performed using a universal test machine (Q-test) at a cross-head speed of 0.5 mm min −1 and K IC was calculated according the following equation:
K I C = 3 P L 2 B W 3 / 2 1.93 a W 1 / 2 − 3.07 a W 3 / 2 + 14.53 a W 5 / 2 − 25.11 a W 7 / 2 + 25.8 a W 9 / 2
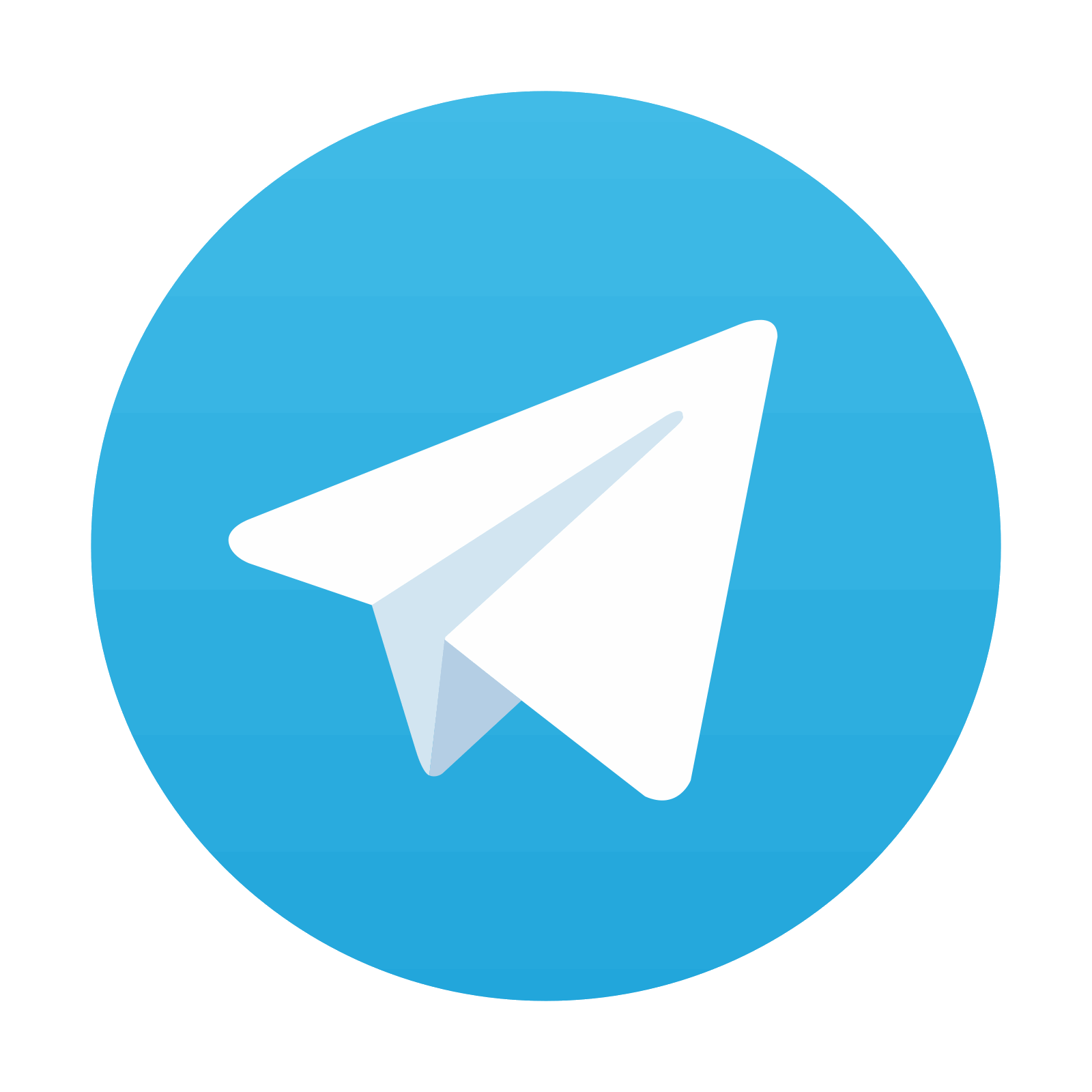
Stay updated, free dental videos. Join our Telegram channel

VIDEdental - Online dental courses
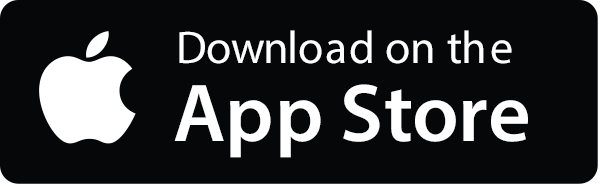
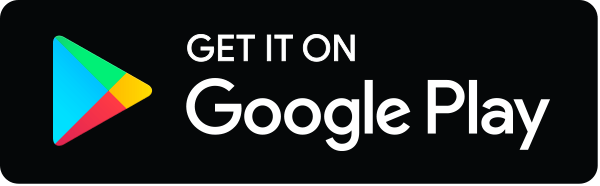