Cellular organization
Publisher Summary
All cells, regardless of the tissue or species from which they are derived, use broadly similar methods to perform such functions as the conversion of chemical bond energy into free energy for cellular processes, the transmission of information from one generation of cells to another, and for the translation of this information into terms of protein structure. Most cells fall within a fairly narrow size range. Thus, the majority of cells have diameters ranging from 0–5 to 20μm. At the lower end of the scale are the bacteria; their size is determined by the number of proteins required to perform the essential functions of the cell and the quantity of DNA required to code for the synthesis of these proteins. The number of different types of protein that are needed has been estimated to be between 500 and 1000, and each of these will require for its specification about 20 times its own weight of DNA. The upper limit of cell size is determined by such physical factors as the ratios of the surface area of the cell membrane to the cytoplasmic volume, and of the nuclear and cytoplasmic volumes. Cells fall into two categories: (1) prokaryotes, which comprise the bacteria and blue-green algae, and (2) eukaryotes, which include protozoal, fungal, plant, and animal cells. In eukaryotic cells, there is a clearly defined nucleus set in what appears under the light microscope to be an amorphous gel, that is, the cytoplasm. Electron microscopy has shown that the cytoplasm is permeated by a network of protein filaments that constitute the cytoskeleton and that it contains a variety of physically and chemically distinct membrane-bound organdies. These include the endoplasmic reticulum, Golgi complex, mitochondria, lysosomes, secretory granules, and peroxisome.
Although the various subcellular structures just described are present in most cells, the degree of their development shows considerable variation in different cell types. Since no single type of cell, e.g. liver, muscle or glandular, can be said to be more typical than another, the features of a hypothetical generalized cell are illustrated in Figure 15.1.
The flow of materials
Membrane components
In aqueous solutions most phospholipids and glycolipids will assemble themselves into double layers in which the polar ends of the molecules are directed outwards and the long fatty acid chains are directed inwards as befits their amphiphilic nature (Figure 8.2b). Bimolecular lipid structures of this type are an essential feature of membrane structure.
Membranes also contain relatively large amounts of cholesterol which is thought to increase the mechanical stability and to help regulate the fluidity of the membrane. The relatively flat steroid ring is inserted between the phospholipid molecules with the polar −OH group adjacent to the polar heads of the phospholipids (Figure 15.2).
The most widely applicable model of membrane structure which allows for the physicochemical properties of membrane lipids and proteins and accounts for the dynamic nature and versatility of membranes is the fluid mosaic model (Figure 15.3). According to this the membrane is composed of a two-dimensional array of phospholipid and globular protein molecules in which the protein molecules are floating in a phospholipid continuum. About 70–80% of the protein molecules are integral proteins, which are held in association with the lipid bilayer by hydrophobic interactions. They, like the lipids, are amphiphilic and form an essential part of the structure. Other proteins known as peripheral or surface proteins can be dissociated from the membrane by the addition of salts. From this it is concluded that they are bound to the membrane surface by hydrogen bonds and salt linkages.
All eukaryotic cells have carbohydrate groupings on their external surface. These are mainly the oligosaccharide side chains of glycoproteins and glycolipids. In addition the ‘cell coat’ may contain secreted glycoproteins and proteoglycans (page 407). The cell surface carbohydrates contain mannose, fucose and sialic acid in addition to glucose, glucosamine, galactose and galactosamine. The sialic acid is usually found at the ends of the side chains which are often branched, and it is the sialic acid which is believed to be mainly responsible for the negative charge found on the surface of all eukaryotic cells. The cell surface carbohydrates play an important role in recognition processes and cellular interactions.
The structure of membrane proteins
Structural studies on integral proteins suggest that the portions of polypeptide chain which are located within the lipid bilayer take up an α-helical conformation. Thus the membrane-crossing regions of these proteins have a relatively rigid rod-like structure. Two structurally distinct classes of integral protein occur. In the first class, which could be described as anchored proteins, the active site of the protein is held in the aqueous environment at the membrane surface while a single helical segment of protein traverses the membrane anchoring the protein within it (Figure 15.4a). Examples of this type of protein are the intestinal hydrolases as well as hormone receptors and antigens such as the histocompatibility antigens on which recognition sites are presented at the membrane surface. The integral proteins which function in membrane transport and as ‘gates’ have a different type of structure. The part within the membrane which forms the transporting region is complex and is made up of a cluster of transmembrane helices (Figure 15.4b). The sodium pump and the calcium pump of muscle are proteins of this type.
Membrane transport and membrane gates
Carrier-mediated transport processes
Carrier-mediated transport can be divided into two types:
1. Passive transport or facilitated diffusion. In this form no energy is expended and movement only takes place from a region of higher to one of lower concentration. However, owing to the presence of the carrier, substances can pass more rapidly along the concentration gradient existing across the membrane than would be expected from simple physicochemical considerations. The uptake of glucose into most cells occurs by passive transport of this sort.
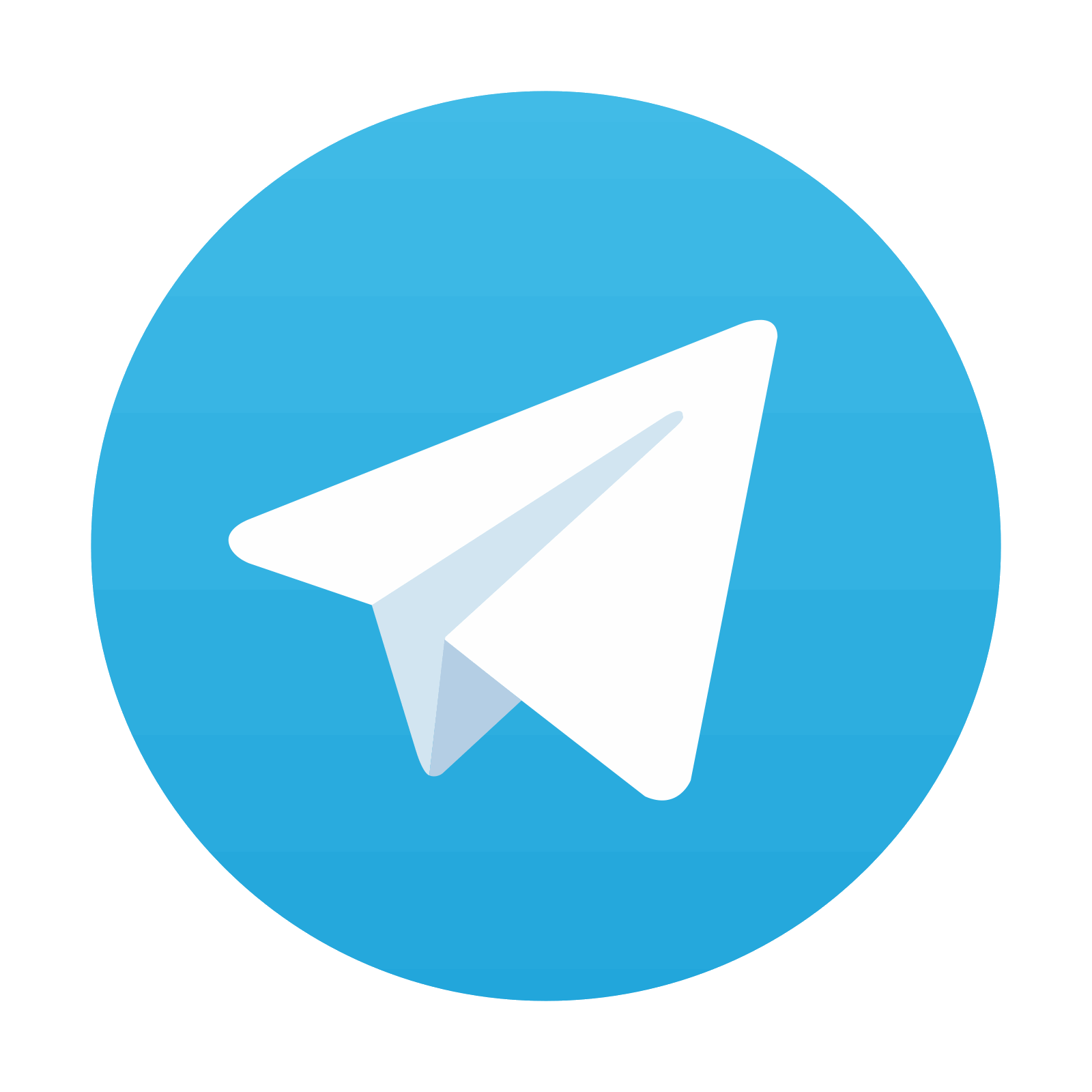
Stay updated, free dental videos. Join our Telegram channel

VIDEdental - Online dental courses
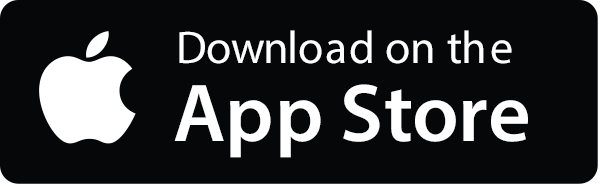
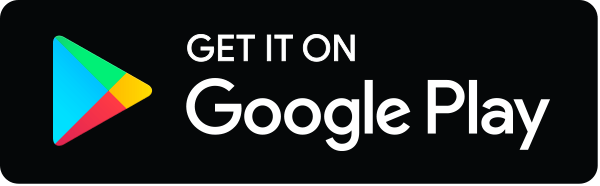