Fig. 11.1
From Li and Neaves [4]. Comparison of stem cell activation during wound healing and carcinogenesis (needs copyright approval)
The third phase of remodeling and maturation occurs between 2 weeks and several months. This process involves production of collagen and proteoglycan from the activated fibroblasts PMID: 24931397. During maturation phase, the fibroblasts disappear from the wound and are replaced by well-organized collagen scaffold.
In addition to the local wound milieu, immunosuppression observed during the postoperative period may contribute to the progression of minimal residual tumors into local recurrence.
In an experimental study in the hamster carcinoma model, surgery has caused increase in the incidence of cancer development. It is also speculated that surgical intervention of oral leukoplakia may increase the incidence of carcinogenesis within the lesion compared to observation [7]. The possible mechanisms of tumor initiation in the field surrounding the tumor, CSC-mediated and otherwise, have been detailed in other chapters (Chapter 1 and Chapter 14).
11.3 Biologic Basis of Tumor Recurrence Following Genotoxic/Cytotoxic Chemoradiotherapy
Recurrence post chemo-radiotherapy, as is the case of surgery, also signifies the escape of the cells from treatment. Although, in this case, the cells escape from the chemotherapy insult by adopting multiple cellular and molecular pathways. Treatment failure post chemo or radiotherapy, is hence, primarily dependent on the underlying molecular profile; a deeper understanding of the mechanisms of drug action and the targeted pathways is mandatory if resistance-response patterns are to be understood. The redundancy of most molecular pathways, a feature adapted to maintain major functions of the body, is also the tool adopted by the cancerous cells to escape the inhibitory effects of cytotoxic or targeted therapy, leading to resistance and treatment failure. An understanding of the major pathways that contribute toward drug resistance may enable selection of patients based on the molecular profile of the patients, activity of the targets, and other associated biomarkers, thereby improving treatment outcome.
Genotoxic and/or cytotoxic therapy forms a major approach toward cancer treatment and along with targeted therapy has contributed towards improving the survival rates of cancers including oral cancer. Nevertheless, drug resistance is a major challenge, and studies down the decades have documented many molecular players that affect the process (Fig. 11.2).

Fig. 11.2
Mechanisms of drug resistance to genotoxic/cytotoxic therapy. The levels at which resistance to cytotoxic therapy is achieved by the cancer cells are elucidated
11.3.1 Molecular Basis of Drug Resistance
Cancer cells adopt varied mechanisms (Fig. 11.2) to evade the cytotoxic action of the chemotherapeutic drugs administered to the patients. These operate at multiple levels that work either individually or in combination toward providing an escape route to the cells from drug action. Resistance to genotoxic drugs is reported to be achieved by several methods such as DNA repair, drug efflux, metabolism and antiapoptotic machinery in addition to other approaches like p53-dependent ER/Golgi pathways, epigenetic modifications of HDAC and transcription of alternative splice forms that enable the cells to escape targeting by the drugs [8].
Uptake and Efflux of the Drug
The primary and first level of resistance mechanisms involves decreasing the uptake of the drug as well as facilitating their increased efflux out of the cell PMID: 25757878, PMID: 2564628. The latter is facilitated by the increased expression of several drug transporters such as the MRPs, ABC family genes and ATP7 PMID: 16815813. In case of cisplatin, the intake controlled by CTR1 while the efflux is regulated by ATP7A and ATP7B, the expression levels of these transporters, hence play a major role in the availability of the drug within the cell.
Alteration of Targets
The next level at which cells can acquire resistance to the drugs that are administered is by alteration of targets; these can be modifications in microtubules/associated proteins (taxanes), thymidylate synthases (5-FU), or specific molecules as in the case of targeted therapy. Taxanes are known to induce polymerization of microtubules and thereby causing a G2/M phase arrest in the cells PMID: 24306928; modifications in tubulin proteins (mutation/expression) itself, regulation of the microtubule-associated proteins (MAPs), and posttranslational modifications of tubulin are known to contribute toward taxane resistance PMID: 22390762, PMID: 18068131, PMID: 11728383.
Deregulation of DNA Repair Pathways
Platinum-based drugs act by generating DNA adducts PMID: 24810023, PMID: 24510227; the effect of this targeted action is nullified due to an increased expression of DNA repair genes PMID: 22659329. XRCC, ERCC and MLH families of genes involved in nucleotide excision repair and are known to be a major causal factor behind cisplatin resistance in the cells PMID: 20189873. DNA polymerases such as POLH and POLB involved in translesional replication, a repair process that facilitates replication past the lesions that are present on the DNA (thymidine dimers), are another class of molecules that can help to alter or bypass the cytotoxic action of the drugs.
Drug Metabolism
Another level of resistance mechanism adopted by the cancer cell is with regard to the drug metabolism by detoxifying enzymes. Glutathione transferases (GST), superoxide dismutases (SOD) and myeloperoxidases are enzymes that regulate the levels of the drugs in the cell thereby contributing toward the resistance of the cell PMID: 25372413.
Anti-apoptotic and Cell Cycle Check Points
The final consequence of the resistance mechanisms adopted by the resistant cell is prevention of apoptosis and continued proliferation. At this level, differential expression of proapoptotic (p53) and antiapoptotic molecules (Bcl-XL) in combination with the cell cycle proteins (CDKs and ATR) further ensures that the cancer cell maintains its resistant property PMID: 14576837, PMID: 17848273, PMID: 16020667.
11.3.2 Markers of Resistance/Response
11.3.2.1 In Vitro Evidence
Studies using cell lines have been invaluable in providing information with regard to the molecular basis of drug resistance in most solid tumors. Studies in HNSCC cell lines have identified several classes of genes as paramount in drug resistance. Global transcript profiling of sensitive and resistant cell lines identified MMP-7 and MMP-13 as important for intrinsic cisplatin sensitivity (ICS) [9]. Correlation of marker profiles between the cisplatin-sensitive and resistant cell lines also indicated a deregulation of markers involved in DNA repair (RECQL, [10]; PMID: 25327479), cell cycle (CCND1, CCND3) [11] and miRNA profiles [12]. Studies in cell lines have implicated other pathways involved in EMT and metastasis (Twist-miR181-a; MACC1, YAP1), glucose transport (GLUT1) and CSC marker (CD147, CD44) [PMIDs: 25846049, 25501015, 24148247, 25421538, 25327479, 23617290, 23413783]. EGFR is another molecule that has been identified to influence the resistance to the TPF regimen in vitro; knockdown of the molecule in cell lines increases sensitivity to the drugs [13]. TPF-resistant cell lines are also known to overexpress markers of cell cycle regulation (survivin), DNA repair (ERCC1) and MDR (MDR1 and PgP-2) [14]. In vitro studies have also revealed the HNSCC cells with varied genetic background may need modified approaches; cells with mutant p53 can be sensitized to cisplatin by inhibition of checkpoint kinases such as Wee-1 [PMID: 25504633] (Tables 11.1 and 11.2).
Table 11.1
Markers of resistance/sensitivity toward chemotherapy in head and neck cancer
Sl No
|
Marker
|
Alteration
|
Drug
|
Resistance
|
Sensitivity
|
Evidence level
|
---|---|---|---|---|---|---|
Mutation
|
||||||
1
|
MMP3
|
1612ins A
|
Cisplatin-5Fu
|
+
|
P
|
|
2
|
p53
|
72R
|
Cisplatin
|
+
|
P
|
|
3
|
Exon2-4
|
NACT
|
+
|
P
|
||
4
|
p53
|
Mutation exon 4–9
|
Platinum
|
+
|
P
|
|
5
|
LOH 17p/9p
|
Platinum, 5-FU
|
+
|
P
|
||
6
|
ERCC1
|
Exon2–4
|
Cisplatin
|
+
|
In vitro
|
|
Expression
|
||||||
10
|
p53
|
High expression protein
|
Platinum
|
+
|
P
|
|
11
|
CCND1
|
High expression
|
Cisplatin
|
+
|
In vitro
|
|
12
|
EGFR
|
High expression
|
TPF
|
+
|
In vitro
|
|
13
|
c-erbB2
|
High expression
|
TPF
|
+
|
P
|
|
14
|
SNAIL
|
High expression
|
Cisplatin
|
+
|
In vitro
|
|
15
|
XPF4, ERCC4
|
High
|
TPF
|
+
|
P
|
|
16
|
Bcl2, XIAP
|
High
|
Platinum
|
+
|
P
|
|
17
|
Pgp, MDR1, MRP
|
High
|
Platinum
|
+
|
P
|
|
18
|
CD44, CD133
|
Expression
|
TPF
|
+
|
P
|
|
20
|
Thymidine phosphorylase
|
Low
|
TPF
|
+
|
P
|
|
21
|
GST-pi
|
High-expression protein
|
Platinum
|
+
|
P
|
|
22
|
Acetylated tubulin
|
High
|
TPF
|
+
|
P
|
|
23
|
GDF15
|
Low
|
TPF
|
+
|
P
|
|
24
|
Annexin
|
Low expression
|
TPF
|
+
|
Patient data
|
|
26
|
miR 100, miR-130a, miR-197
|
Low
|
Cisplatin
|
+
|
In vitro
|
|
27
|
miR-101m, miR-181b, miR-181d, miR-195
|
High expression
|
Cisplatin
|
In vitro
|
||
28
|
miR34a
|
Downregulation
|
Cisplatin
|
+
|
Patient data
|
Table 11.2
Markers of resistance/sensitivity toward targeted chemotherapy in head and neck cancer
Sl No
|
Marker
|
Alteration
|
Drug
|
Resistance
|
Sensitivity
|
Evidence level
|
---|---|---|---|---|---|---|
EGFR inhibitors
|
||||||
1
|
EGFR-T790M, T590M
|
Mutation exon 20
|
Cetuximab
|
+
|
In vitro, In vivo
|
|
2
|
EGFR-T790M, T590M
|
Mutation exon 20
|
TKI
|
+
|
In vitro, in vivo
|
|
3
|
KRAS
|
p.Gly12Val
|
Cetuximab + RT
|
+
|
Patient data
|
|
4
|
G2607A
|
Mutation exon 20
|
TKI
|
+
|
Patient data
|
|
5
|
PP2A
|
Low
|
Cetuximab
|
+
|
In vitro
|
|
6
|
CIP2A
|
Overexpressed
|
Cetuximab
|
+
|
In vitro
|
|
7
|
NF1
|
Low
|
Cetuximab
|
+
|
In vitro
|
|
8
|
DUSP5
|
Low
|
Cetuximab
|
+
|
In vitro
|
|
9
|
VEGF, IL6
|
High (serum)
|
Cetuximan + platinum/taxol
|
+
|
Patient data
|
|
10
|
p21
|
High
|
Erlotinib
|
+
|
Patient data
|
|
COX–2 inhibitors
|
||||||
1
|
PGE2
|
High expression
|
NSAIDS
|
+
|
Patient data
|
|
2
|
PPAR-gamma
|
Low
|
NSAIDS
|
+
|
Patient data
|
|
3
|
EGFR
|
High expression
|
Celecoxib + erlotinib
|
+
|
In vitro + patient data
|
|
4
|
pERK
|
High expression
|
Celecoxib + erlotinib
|
+
|
In vitro + patient data
|
|
5
|
pS6
|
High expression
|
Celecoxib + erlotinib
|
+
|
In vitro + patient data
|
|
6
|
pAKT
|
High expression
|
Celecoxib + erlotinib
|
+
|
In vivo and in vitro
|
|
7
|
pSTAT3
|
High expression
|
Celecoxib + erlotinib
|
+
|
In vivo and in vitro
|
|
mTOR inhibitors
|
||||||
1
|
PTEN
|
Knockdown
|
Rapamycin
|
+
|
In vitro data
|
|
2
|
VEGF
|
High expression
|
Rapamycin + erlotinib
|
+
|
Patient data
|
|
VEGFR1, IGN-gamma
|
High expression
|
Sirolimus + erlotinib
|
+
|
Patient data
|
||
Bcl-2, MDR1
|
Absence
|
Rapamycin
|
+
|
In vitro data
|
11.3.2.2 Correlation of Molecular Evidence with Survival and Prognosis
In vitro studies are extremely valuable in terms of delineating the underlying mechanisms of drug resistance; nevertheless, assessment of patient-related data and clinical trials have provided essential information with regard to the correlation between the markers and treatment response. These studies have emphasized on the prognostic role of sequence based alterations, expression of transcripts/proteins regulating cell cycle, apoptosis and DNA repair.
Alterations in p53, one of the most prevalent in HNSCC, as per The Cancer Genome Atlas (TCGA), have been identified as prime in resistance to drug. Assessment of loss of heterozygosity (LOH) and microsatellite instability (MSI) in HNSCC patients, identified LOH at 9p or 17p (location of p53) to be significantly associated with drug resistance, LOH at 17p being predictive of low response to platinum-5-FU chemotherapy [15]. Mutations in exons 4–9 of p53 were of high prevalence in HNSCC (~68 %) with these patients showing no response to neoadjuvant therapy [16], mutations being more specifically predictive of response in patients with laryngeal [17] and maxillary squamous cell cancers [18]. Alterations in the codon 72 of p53, encoding either arginine (72R) or proline (72P), effect response to cisplatin; patients with 72R showing extremely low response rates [19].
Expression of p53 (at protein level) in combination with thymidylate synthase (TS) and GST-pi also significantly correlated with response and survival to cisplatin-based neo-adjuvant chemotherapy NACT [20]. Immunohistochemical levels of GSTs when scored against chemotherapy response of patients showed that overall response rate (CR + PR) in patients with low GST was 88 %, while those with high GST scores only showed 19 % response (p = 0.0001). Among the subset treated with NACT, 100 % response was observed in GST low patients, while in patients treated with chemotherapy for relapsed disease, the response rate was 70 % [21]. When the genes involved in thymidine synthesis and metabolism were assessed for their association with response to combination therapy (radiation with induction CT of platinum/5-FU or concurrent platinum therapy), it was observed that lesser percentage of cells with nuclear thymidine phosphorylase (TP) in pretreatment biopsies was associated with high rate of complete response (CR). These patients also showed a high relapse-free survival (p = 0.001) [22].
Multidrug resistance proteins, MDR1 and MRP, are associated with resistance to therapy in oral cancer; expression of these proteins was high in the nonresponders as compared to responders in patients with tongue cancer [23]. Another study reported the induction of PgP by radiation and may explain the low response rates of patients who are subjected to concurrent chemotherapy [24]. Assessment of patients with advanced cancers of the head and neck also showed that nonresponders had a high expression of PgP and MDR-3 [25].
Markers of DNA repair are extensively correlated to treatment response; studies associating response to molecular profile in patients showed that increased co-expression of SNAIL and ERCC1 (SNAIL is a known activator of ERCC1) contributes toward resistance to cisplatin and was also found to correlate with prognosis in patients with HNSCC [26]. Expression of ERCC1 along with Bcl-2 and other multidrug-resistant genes (MDR-1, MRP-1 and ATP7B) were downregulated in recurrent maxillary disease [18]. Analysis of ERCC1 alterations at mutation, protein and transcript levels indicated an increased association with treatment outcome. In patients treated with adjuvant cisplatin-based chemoradiation, high IHC scores (H-score) of ERCC1 protein correlated with response and survival [27]. Further, in patients treated with cisplatin-based induction therapy followed by concurrent chemoradiation, low immunohistochemical levels of ERCC1 correlated with increased 12-month disease-free (73.3 % vs. 42.3 %, p < 0.001) and overall survival [28]. Other members of the ERCC family, XPF, or ERCC4 levels in IHC are also shown to correlate with progression-free survival in patients treated with DNA-damaging agents [29].
Deregulation of cell cycle and apoptotic proteins plays a major role in carcinogenesis as well as resistance to drugs. In patients exposed to neoadjuvant therapy (cisplatin based), low CCND1 expression indicated better response (p < 0.0001) with significantly better OS and DFS (73 % vs. 8 %, p < 0.001; 63 % vs. 6 %, p < 0.001); there was no correlation in patients treated with surgery and radiation indicating that this marker might be predictive in selecting patients for NACT [30]. Tumors treated with cisplatin, positive for the apoptotic protein, Bcl-2, prior to treatment had a high risk of treatment failure with high hazard ratio (hazard ratio, 5.99; 95 % confidence interval, 1.73–20.8; P = 0.0014) [31]. Studies have also shown increased expression of the Bcl-XL to correlate with low DFS in patients with advanced disease [25]. X-linked inhibitor of apoptosis (XIAP) was also associated with resistance to cisplatin (p = 0.036) and outcome. Additionally, it was observed that the expression of this gene was further induced in patients exposed to the therapy, which lead to a poorer outcome in this subset [32].
Alterations in the targets of the cytotoxic agents are a major mechanism of drug resistance adopted, especially in cases of taxanes. Increased expression of acetylated tubulin is known to correlate with occurrence of lymph node metastasis in HNSCC patients treated with docetaxel along with cisplatin and 5-FU. Extensive evidence correlating tubulin modifications with taxane resistance is available in other cancers; β-tubulin mutations in serum DNA were associated with paclitaxel resistance in non-small cell lung cancer (NSCLC) [33].
Many other markers have shown correlation with treatment response; polymorphisms in matrix metalloproteinase 3 (1612insA) promoter; 6A/6A genotype (wherein both the alleles have 6 adenosines at the position due to the insertion) was an independent response factor for patients treated with 5-FU-cisplatin therapy as compared to 5A/6A (heterozygous with one allele having no insertion) or 5A/5A genotypes (wild type allele with no insertions at the position) [34]. Other studies in patients, treated with 5-FU or cisplatin and paclitaxel, have shown increased expression of c-erbB2 to correlate with progression-free and overall survival [35]. Among other markers, downregulation of miR34a, involved in regulation of silent information regulator 1 (SIRT1) and thereby p53, was associated with poor DFS and local control rates in HNSCC patients treated with cisplatin [36] (Fig. 11.3).

Fig. 11.3
Cytotoxic Therapy markers: Markers associated with resistance and response to cytotoxic therapy in head and neck and oral cancer markers identified in HNSCC to be associated with treatment response and prognosis are listed
The TPF combination therapy regime is considered as the gold standard of induction chemotherapy in head and neck cancer, but resistance to this regime has also been a major challenge. In patients with clinically positive nodes (clinically node positive, cN+), high cyclin D1 expression was shown to predict benefit from addition of TPF regimen to standard treatment [37]. Expression of growth-dependent factor (GDF15) is shown to correlate with poor prognosis in patients treated with TPF therapy [38]. Low GDF predicts good overall as well as distant metastasis-free survival in these patients. Other studies in TPF-treated patients, evaluating marker correlation with response, indicated that acetylated tubulin (AT), annexin (low) and CDK1 (high) were predictors of response [39, 40]. In advanced recurrent patients treated with taxol and platinum, low levels of ERCC1 and increased RASSF1A were predictive of good prognosis [41]. Molecular profiling of oropharyngeal patients in the TAX324 trial (comparing TPF against PF in locally advanced HNSCC) showed that low beta tubulin II (beta T-2) was predictive of therapy benefit [42]. Categorization of the oropharyngeal patients of this trial based on their HPV status indicated that the presence of a specific molecular profile (high beta T-II, GST-pi and p53 along with low Bcl-2) was predictive of survival rates when treated with TPF/PF [43]. This observation emphasized the significance of risk stratification prior to treatment selection. A concept further gaining significance is the role of cancer stem cells in imparting drug resistance in cancers including head and neck cancer; studies have shown that in patients treated with the TPF regimen of drugs, expression levels of CD44, BMI1 and Notch1 correlated with recurrence. In resistant patients, a consequent enrichment of these markers posttreatment is a further indicator of poor response [44]. This aspect of the CSC-mediated drug resistance has been discussed in detail in the chapter 14.
11.4 Resistance to Targeted Therapy
Targeted therapies currently under investigation in HNSCC include anti-EGFR therapy (cetuximab), anti-VEGF (bevacizumab), and anti-COX-2 (celecoxib) [45–51]. Despite the advantage of targeting molecules/their alterations highly prevalent in head and neck cancer and being extremely specific to the cancer cells, these targeted therapies, in most cancers, including HNSCC fail to provide high rates of response in the patients. Unlike in the case of cytotoxic chemotherapy, in these cases, the resistant mechanisms are more pathway-specific and thereby unique to each targeted therapy.
11.4.1 Anti-EGFR Therapy
11.4.1.1 EGFR Pathway
EGFR, 170 KDa cell surface receptor protein with extracellular ligand binding and an intracellular domain with tyrosine kinase activity [47], aids in the normal development and proliferation of epithelial tissue and is found to be overexpressed in 80–90 % of head and neck cancer. EGFR overexpression, correlated with reduced survival, poor prognosis, and aggressive disease [52], is reported to be responsible for cell proliferation, differentiation, antiapoptotic signaling, angiogenesis and metastasis in various malignancies. EGFR is activated through ligand binding which leads to autophosphorylation of the tyrosine kinase domain. The various EGFR ligands are TGF-α, amphiregulin, epiregulin, EGF, epigen, betacellulin and heparin-binding EGFR. The EGFR pathway has different signaling routes: P13K-PDK1-AKT, Ras-Raf-MEK-ERK (mediates transcription and activates other proteins), PLCγ-PKC (cell cycle progression), and JAK-STAT (cell proliferation, survival, transformation) [53, 54] (Fig. 11.4).

Fig. 11.4
EGFR signaling routes and the resulting downstream processes (From Loeffler-Ragg et al. [47])
11.4.1.2 Markers of Resistance to Anti-EGFR Therapy
Targeting EGFR pathway, in patients wherein it is dysregulated, is hence considered a viable therapeutic option due to its increased prevalence in the patients as well it is functional relevance. The approaches towards EGFR targeting includes i) blockage of ligand binding using monoclonal antibodies (Cetuximab and Panitumumab) ii) blockage of its TKI domain (Geftinib, Erlotinib) activity thereby blocking the downstream signaling molecules. Nevertheless, the response rates in patients to these various therapies ranges between 10–40%, either due to primary or acquired resistance. The possible resistance mechanisms for EGFR therapy include mutation of EGFR, oncogenic shift or activation of a bypass pathway, and/or due to modification of any pathway/molecule essential for EGFR TKI-mediated apoptosis [55] (Fig. 11.5).

Fig. 11.5
Markers of resistance and response to EGFR therapy. The primary EGFR pathway is shown in addition to the associated molecules that might be involved in resistance to the drug. The markers with clinical evidence are indicated in red
Targeting the EGFR protein is primarily through the use of monoclonal antibodies; Cetuximab and panitumumab are used for blocking the ligand binding to the receptor; they bind with a higher affinity as compared with the regular ligands. Cetuximab binds specifically to the extracellular domain [56]. Correlation of the marker profile with response to the drug indicated that expression of EFGR protein was indicative of response in the trials such as E2303 [Eastern Cooperative Oncology Group (ECOG)], Erbitux in First-Line Treatment of Recurrent or Metastatic Head and Neck Cancer (EXTREME), and Cetuximab combined with Irinotecan in first line therapy for metastatic colorectal cancer(CRYSTAL) carried out in patients [57, 58]. Further assessment of the patients identified extracellular ERK1/2 and activated RAS/MAPK/ERK and/or PI3K/AKT pathways as indicative of PFS and OS [59]. However, marker evaluation in EXTREME trial patients showed no association of EGFR copy number with response in metastatic patients [60, 61]. Studies also revealed that serum biomarkers (VEGF, IL-6) were associated with response to cetuximab combination therapy with platinum/taxol) [62]. KRAS mutation (p.Gly12Val) and somatic EGFR mutation located in exon 19 is also suggested to contribute to the limited clinical response to cetuximab therapy in combination with radiotherapy; in the same study, high EGFR expression was indicative of increased treatment response [63]. Additionally, mutation in the 3′ UTR of KRAS have been associated with treatment response; patients (recurrent/metastatic HNSCC with mutation in 3′ UTR KRAS) showed improved response when treated with cetuximab and platinum as compared to cisplatin treatment alone [64].
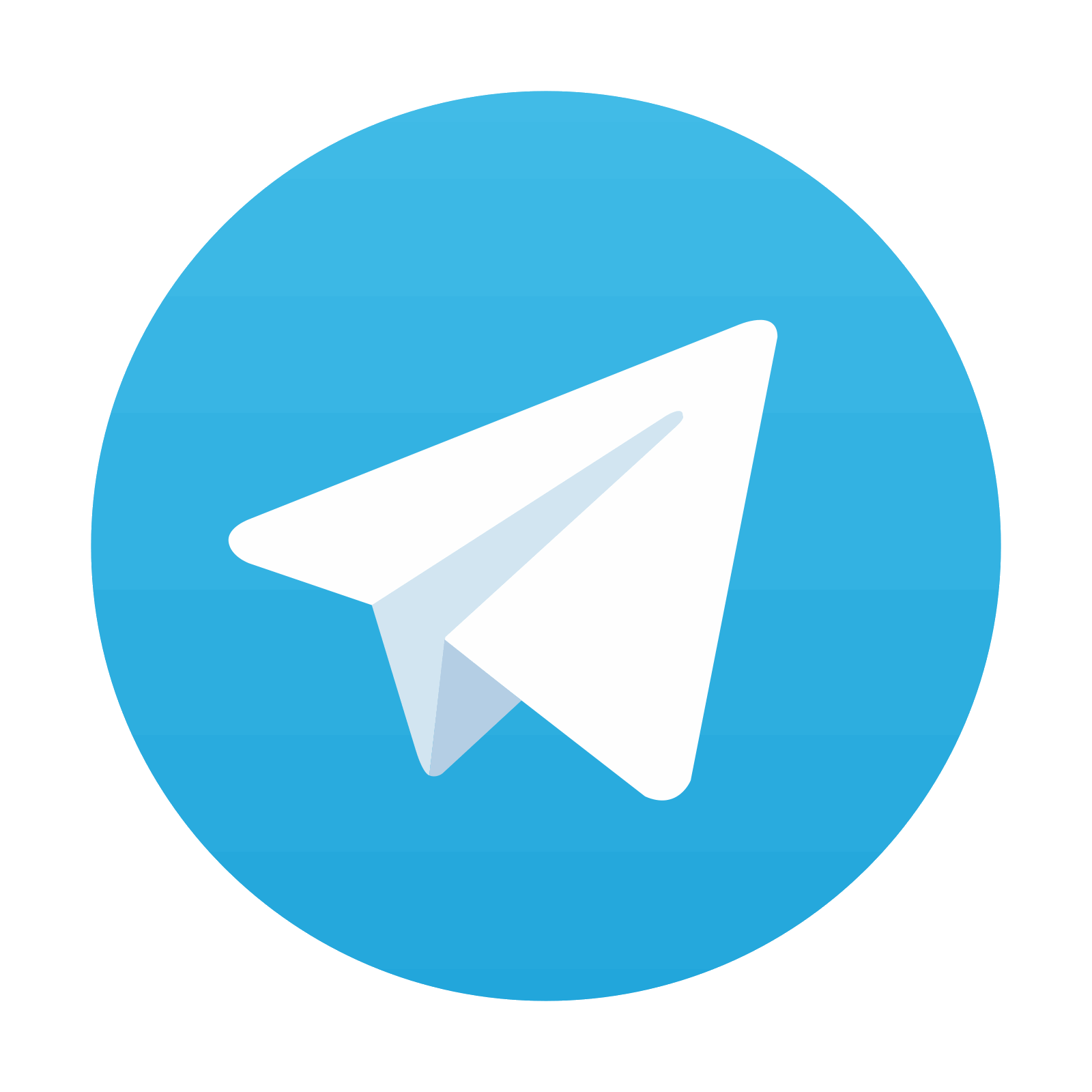
Stay updated, free dental videos. Join our Telegram channel

VIDEdental - Online dental courses
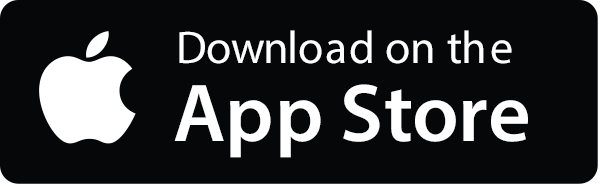
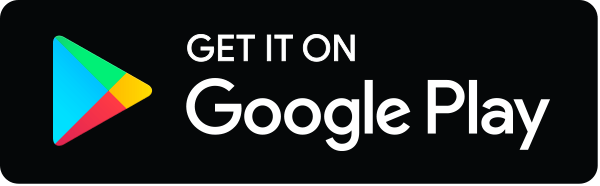