Highlights
- •
We compared adherence of oral Streptococci to titanium nanotubules.
- •
We showed that adherence increased with nanotubule diameter.
- •
Adherence was also correlated with surface fluoride content.
- •
Adherence of oral streptococci can be modified by titanium anodization.
Abstract
Objectives
Peri-implantitis and peri-mucositis pose a severe threat to the success of dental implants. Current research focuses on the development of surfaces that inhibit biofilm formation while not inferring with tissue integration. This study compared the adherence of two oral bacterial species, Streptococcus sanguinis and Streptococcus mutans to nanostructured titanium surfaces.
Methods
The samples included TiO 2 nanotubes formed by anodization of titanium foil of 100, 50 and 15 nm diameter (NT15, NT50, NT100), a nanoporous (15 nm pore diameter) surface and compact TiO 2 control. Adherent surviving bacteria were enumerated after 1 h in an artificial saliva medium containing bovine mucin.
Results
Lowest numbers of adherent bacteria of both species were recovered from the original titanium foil and nanoporous surface and highest numbers from the Ti100 nanotubes. Numbers of attached S. sanguinis increased in the order (NT15 < NT50 < NT100), correlated with increasing percentage of surface fluoride. The lowest adhesion of S. sanguinis and S. mutans on TiO 2 nanostructured surfaces was observed for small diameter nanoporous surfaces which coincides with the highest osteoblast adhesion on small diameter nanotubular/nanoporous surfaces shown in previous work.
Significance
This study indicates that the adherence of oral streptococci can be modified by titanium anodization and nanotube diameter.
1
Introduction
The reported success rates of dental implants are high when considered solely in terms of initial implant osseointegration, however there is unpredictability in the initial generation and subsequent stability of the peri-implant soft tissue architecture over the lifetime of the implant . The adherence and proliferation of potentially pathogenic bacteria on Ti implant surfaces has been directly linked to the peri-implant inflammatory changes which occur and may be limited to the soft-tissues (mucositis) or associated with progressive loss of osseointegration (peri-implantitis) . The results of a review of the frequency of peri-implant diseases involving a meta-analysis of 504 studies with 1497 participants and 6283 implants suggested that 63.4% of the participants and 30.7% of implants suffered from peri-implant mucositis and 18.8% participants and 9.6% of implants were affected by peri-implantitis . As the number of dental implants increases in the population, peri-implant disease is likely to become a particularly significant burden for patients and healthcare providers alike. This is of particular significance in the elderly where a loss of manual dexterity and a decreased salivary flow predisposes to poor hygiene around dental implant fixtures leading to an increased risk of peri-implant disease . Consequently, contemporary research is focusing on the development of surfaces to facilitate osseointegration , as well as to support the formation of a healthy cuff of keratinized mucosa around the implant abutment, providing a barrier to prevent the passage of microorganisms into the underlying connective tissues .
Nanotexturing is being widely explored as a means of encouraging cellular attachment and techniques include the incorporation of nanoparticulates and modification of nanoscale surface topography to encourage cell adhesion and inhibit bacterial attachment and biofilm formation .
In the past, several methods have been developed to produce nanoscale structures on Ti surfaces and electrochemical anodization of Ti is a powerful tool to control nanoscale architecture for surface modification. To improve the biological, chemical, and mechanical properties of the biomaterial, significant research has been carried out to find more suitable topologies which could present improved bioperformance (reviewed by Kulkarni et al. ). Titanium dioxide (TiO 2 ) nanotubes can be easily synthesized by electrochemical anodization of Ti using a two electrode electrochemical cell, and the electrochemical formation of self-organized TiO 2 nanotubes layers in dilute fluoride containing electrolytes has been studied intensively . It was shown that the water content in the electrolyte is the critical factor that determines whether self-ordered oxide nanotubes or nanopores are formed during the electrochemical anodization, and it is thought that tube formation originates from ordered porous oxide by a “pore-wall-splitting” mechanism . By tailoring the anodization parameters (applied voltage, anodization time and concentrations of chemicals) TiO 2 nanotubes of different diameters from 15 nm to 300 nm and different lengths can be obtained . Osteoblast adhesion to TiO 2 nanotubes has been shown to be dependent on tube diameter . Peng et al. found that osteoblast adhesion was enhanced, and Staphylococcus epidermidis adhesion and proliferation reduced on 80 nm compared with 30 nm diameter TiO 2 tubules and smooth controls. They concluded that the effect was due to a combination of surface chemistry (fluorine content), surface roughness and wettability. Gongadze, et al. similarly reported that osteoblasts adhered better to 15 nm diameter nanopores/nanotubes than 100 nm diameter nanotubes . However, the effect of such surface modifications on attachment of oral bacteria has not been reported. In the present work, the effect of different nanostructured Ti surface on the adherence of two oral Streptococcal species, S. sanguinis and Streptococcus mutans that coexist in oral biofilms was investigated. S. sanguinis is a primary colonizer of oral plaque and although S. mutans is primarily associated with dental caries, both species have been identified in association with dental implants .
2
Materials and methods
2.1
Preparation of Ti nanotextured surfaces
Ti foils of 0.1 mm thickness and 99.6% purity were used for preparation of different nanostructures. Prior to two-step anodization, Ti foils were degreased by successive ultrasonication in acetone, ethanol and deionized (DI) water for 5 min each and dried in a nitrogen stream. The two-steps anodization (leading to a pre-patterning of the surface) was carried out to achieve nanostructures with higher uniformity and less defects (as a result of using the pre-patterned substrate in the second anodization). All anodization experiments were performed at room temperature, in a two-electrode system with Ti foil as the anode and platinum gauze as the cathode, with a working distance of 15 mm. Briefly, in the first step, Ti foils were anodized in ethylene glycol based electrolyte with 1 M H 2 O and 0.1 M NH 4 F at 35 V for 2 h. Nanotubes grown in this first step were removed by ultrasonication to get a pre-patterned surface which was then cleaned by successive ultrasonication in acetone and ethanol and used as a substrate for the second step . In the second step, nanostructures were grown in ethylene glycol (EG) based electrolytes containing hydrofluoric (HF) acid and water (H 2 O) with specific chemical compositions as shown in Table 1 . After the anodization, the nanostructures formed were immersed in ethanol for 2 h to remove all organic components from the electrolyte, washed with distilled water and dried in a nitrogen stream.
Ti surface/NP/NT diameter | Electrolyte | Potential used | Anodization time |
---|---|---|---|
Compact oxide | 1 M phosphoric acid | 20 V | 15 min |
NP 15 nm | EG + 6 M water + 0.2 M HF | 10 V | 1 h |
NT 15 nm | EG + 8 M water + 0.2 M HF | 10 V | 2.5 h |
NT 50 nm | EG + 8 M water + 0.2 M HF | 20 V | 2.5 h |
NT 100 nm | EG + 8 M water + 0.2 M HF | 58 V | 2.5 h |
2.2
Surface characterization of Ti nanostructures
The morphology of the TiO 2 nanostructures was observed using a field-emission scanning electron microscope – Hitachi FE-SEM S4800 operating at an accelerating voltage of 10.00 kV at 8 mm working distance.
2.3
Atomic Force Microscopy (AFM)
Topographic features of Ti-foil and Ti-nanostructured surfaces were measured by atomic force microscopy (AFM, Solver PRO, NT-MDT, Russia). All the measurements were conducted in tapping mode in air. Samples were scanned with the standard Si cantilever with a force constant of 22 N/m and at a resonance frequency of 325 kHz (using a tip radius of 10 nm and a tip length of 95 μm).
2.4
XPS Analysis of the surfaces
XPS analysis was conducted by Midlands Surface Analysis Ltd, Aston University, Birmingham, in a Thermofisher ESCALAB 250 electron spectrometer equipped with a hemispherical sector energy analyzer. A monochromatic Al Kα X-ray source was used for analysis to enhance the resolution. At source excitation energy of 15 KeV, an emission current of 6 mA; an analyzer pass energy of 20 eV with step size of 0.1 eV and a dwell time of 50 ms were used throughout the experiments. The base pressure within the spectrometer during examinations always exceeded 5 × 10 −10 mbar, ensuring that all signals recorded were from the sample surface. The area of analysis was 500 μm diameter. XPS survey scans were first recorded for each region examined and then narrow region energy scans were performed for all the elements identified on the surface to determine the chemical state of the specific element identified. To improve statistics, multiple scans were used throughout for all the constituents in the surface.
2.5
Surface wettability
Contact angle measurements were obtained with a Digidrop contact angle meter (GBX, Digidrop Romans Sur Isère, Drôme, France). HPLC grade distilled water ( Y = 72.8 mN m −1 ) 2–2.5 μL droplets were deposited onto each sample surface at room temperature. Contact angles of five drops per sample on 3 samples were analyzed and results are expressed as mean ± SD of the 15 measurements.
2.6
Bacterial adhesion
A fresh colony of S. sanguinis GW2 or S. mutans 3209 was used to inoculate 10 ml of artificial saliva and was then incubated with agitation for 24 h in an anaerobic work station (Mini-MAC, Don Whitley Scientific) at 37 °C in an atmosphere of 10% carbon dioxide, 80–85% nitrogen and 5–10% hydrogen for 24 h. Following vortex mixing to disperse aggregates, the suspension was diluted 100 fold in fresh artificial saliva to give approximately 2 × 10 7 colony forming units (cfu)/ml. Two 5 μl aliquots of the diluted suspension were pipetted onto a sterilized microscope slide enclosed inside a Petri dish to maintain humidity, and the Ti samples to be tested were placed face-down onto the suspension ( Fig. 1 ). The samples were incubated for 1 h in the anaerobic work-station to allow bacterial attachment. Four samples of each Ti surface condition were used for each bacterium. Following incubation the samples were rinsed by immersion in PBS three times to remove loosely bound bacteria. Samples were then placed in separate 7.0 ml bijou tubes (50 mm × 20 mm) containing 5 ml of sterile PBS. The containers were then placed in a sonic water bath (Vitasonic, Bad Säckingen, Switzerland) for 10 min, and vortex mixed for 15 s to detach the bacteria that had adhered to the surfaces. 0.1 ml of the sonicated and vortexed sample was plated onto blood agar plates (BioMérieux, Hampshire, UK), and incubated for 24 h in an anaerobic cabinet to obtain colony counts.

2.7
Statistical analysis
Results were analyzed using Analysis of Variance followed by post-hoc Tukey tests for multiple comparisons.
2
Materials and methods
2.1
Preparation of Ti nanotextured surfaces
Ti foils of 0.1 mm thickness and 99.6% purity were used for preparation of different nanostructures. Prior to two-step anodization, Ti foils were degreased by successive ultrasonication in acetone, ethanol and deionized (DI) water for 5 min each and dried in a nitrogen stream. The two-steps anodization (leading to a pre-patterning of the surface) was carried out to achieve nanostructures with higher uniformity and less defects (as a result of using the pre-patterned substrate in the second anodization). All anodization experiments were performed at room temperature, in a two-electrode system with Ti foil as the anode and platinum gauze as the cathode, with a working distance of 15 mm. Briefly, in the first step, Ti foils were anodized in ethylene glycol based electrolyte with 1 M H 2 O and 0.1 M NH 4 F at 35 V for 2 h. Nanotubes grown in this first step were removed by ultrasonication to get a pre-patterned surface which was then cleaned by successive ultrasonication in acetone and ethanol and used as a substrate for the second step . In the second step, nanostructures were grown in ethylene glycol (EG) based electrolytes containing hydrofluoric (HF) acid and water (H 2 O) with specific chemical compositions as shown in Table 1 . After the anodization, the nanostructures formed were immersed in ethanol for 2 h to remove all organic components from the electrolyte, washed with distilled water and dried in a nitrogen stream.
Ti surface/NP/NT diameter | Electrolyte | Potential used | Anodization time |
---|---|---|---|
Compact oxide | 1 M phosphoric acid | 20 V | 15 min |
NP 15 nm | EG + 6 M water + 0.2 M HF | 10 V | 1 h |
NT 15 nm | EG + 8 M water + 0.2 M HF | 10 V | 2.5 h |
NT 50 nm | EG + 8 M water + 0.2 M HF | 20 V | 2.5 h |
NT 100 nm | EG + 8 M water + 0.2 M HF | 58 V | 2.5 h |
2.2
Surface characterization of Ti nanostructures
The morphology of the TiO 2 nanostructures was observed using a field-emission scanning electron microscope – Hitachi FE-SEM S4800 operating at an accelerating voltage of 10.00 kV at 8 mm working distance.
2.3
Atomic Force Microscopy (AFM)
Topographic features of Ti-foil and Ti-nanostructured surfaces were measured by atomic force microscopy (AFM, Solver PRO, NT-MDT, Russia). All the measurements were conducted in tapping mode in air. Samples were scanned with the standard Si cantilever with a force constant of 22 N/m and at a resonance frequency of 325 kHz (using a tip radius of 10 nm and a tip length of 95 μm).
2.4
XPS Analysis of the surfaces
XPS analysis was conducted by Midlands Surface Analysis Ltd, Aston University, Birmingham, in a Thermofisher ESCALAB 250 electron spectrometer equipped with a hemispherical sector energy analyzer. A monochromatic Al Kα X-ray source was used for analysis to enhance the resolution. At source excitation energy of 15 KeV, an emission current of 6 mA; an analyzer pass energy of 20 eV with step size of 0.1 eV and a dwell time of 50 ms were used throughout the experiments. The base pressure within the spectrometer during examinations always exceeded 5 × 10 −10 mbar, ensuring that all signals recorded were from the sample surface. The area of analysis was 500 μm diameter. XPS survey scans were first recorded for each region examined and then narrow region energy scans were performed for all the elements identified on the surface to determine the chemical state of the specific element identified. To improve statistics, multiple scans were used throughout for all the constituents in the surface.
2.5
Surface wettability
Contact angle measurements were obtained with a Digidrop contact angle meter (GBX, Digidrop Romans Sur Isère, Drôme, France). HPLC grade distilled water ( Y = 72.8 mN m −1 ) 2–2.5 μL droplets were deposited onto each sample surface at room temperature. Contact angles of five drops per sample on 3 samples were analyzed and results are expressed as mean ± SD of the 15 measurements.
2.6
Bacterial adhesion
A fresh colony of S. sanguinis GW2 or S. mutans 3209 was used to inoculate 10 ml of artificial saliva and was then incubated with agitation for 24 h in an anaerobic work station (Mini-MAC, Don Whitley Scientific) at 37 °C in an atmosphere of 10% carbon dioxide, 80–85% nitrogen and 5–10% hydrogen for 24 h. Following vortex mixing to disperse aggregates, the suspension was diluted 100 fold in fresh artificial saliva to give approximately 2 × 10 7 colony forming units (cfu)/ml. Two 5 μl aliquots of the diluted suspension were pipetted onto a sterilized microscope slide enclosed inside a Petri dish to maintain humidity, and the Ti samples to be tested were placed face-down onto the suspension ( Fig. 1 ). The samples were incubated for 1 h in the anaerobic work-station to allow bacterial attachment. Four samples of each Ti surface condition were used for each bacterium. Following incubation the samples were rinsed by immersion in PBS three times to remove loosely bound bacteria. Samples were then placed in separate 7.0 ml bijou tubes (50 mm × 20 mm) containing 5 ml of sterile PBS. The containers were then placed in a sonic water bath (Vitasonic, Bad Säckingen, Switzerland) for 10 min, and vortex mixed for 15 s to detach the bacteria that had adhered to the surfaces. 0.1 ml of the sonicated and vortexed sample was plated onto blood agar plates (BioMérieux, Hampshire, UK), and incubated for 24 h in an anaerobic cabinet to obtain colony counts.
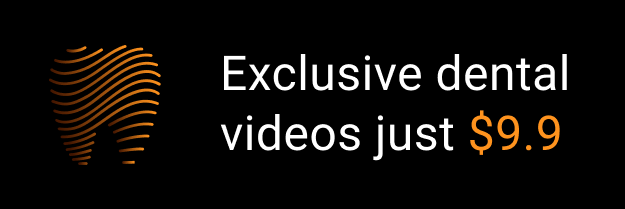