Abstract
Objectives
The majority of modern resin-based oral restorative biomaterials are cured via photopolymerization processes. A variety of light sources are available for this light curing of dental materials, such as composites or fissure sealants. Quartz-tungsten-halogen (QTH) light curing units (LCUs) have dominated light curing of dental materials for decades and are now almost entirely replaced by modern light emitting diode light curing units (LED LCUs). Exactly 50 years ago, visible LEDs were invented. Nevertheless, it was not before the 1990s that LEDs were seriously considered by scientists or manufactures of commercial LCUs as light sources to photopolymerize dental composites and other dental materials. The objective of this review paper is to give an overview of the scientific development and state-of-the-art of LED photopolymerization of oral biomaterials.
Methods
The materials science of LED LCU devices and dental materials photopolymerized with LED LCU, as well as advantages and limits of LED photopolymerization of oral biomaterials, are discussed. This is mainly based on a review of the most frequently cited scientific papers in international peer reviewed journals. The developments of commercial LED LCUs as well as aspects of their clinical use are considered in this review.
Results
The development of LED LCUs has progressed in steps and was made possible by (i) the invention of visible light emitting diodes 50 years ago; (ii) the introduction of high brightness blue light emitting GaN LEDs in 1994; and (iii) the creation of the first blue LED LCUs for the photopolymerization of oral biomaterials. The proof of concept of LED LCUs had to be demonstrated by the satisfactory performance of resin based restorative dental materials photopolymerized by these devices, before LED photopolymerization was generally accepted. Hallmarks of LED LCUs include a unique light emission spectrum, high curing efficiency, long life, low energy consumption and compact device form factor.
Significance
By understanding the physical principles of LEDs, the development of LED LCUs, their strengths and limitations and the specific benefits of LED photopolymerization will be better appreciated.
“ All truth passes through three stages. First, it is ridiculed. Second, it is violently opposed. Third, it is accepted as being self-evident. ” Arthur Schopenhauer
1
Introduction
The introduction of resin-based dental materials near the middle of the last century was a revolution in restorative dentistry. Dental composites are esthetically pleasing since they possess a tooth-like appearance, are stable within the oral environment, are relatively easy to handle and set on command via self curing or light curing.
Today, almost all commercial dental composites utilize photopolymerization reactions initiated by blue visible light. Light curing units (LCUs) based on different physical principles, such as quartz-tungsten-halogen (QTH) bulbs, laser, plasma arc lights, and light emitting diodes (LEDs) are available. Nevertheless, LED LCUs are currently the standard devices in most modern dental practices.
In many cases, clinicians using LED LCUs on a daily basis are unaware of the physics and/or history of their development. This knowledge, however, is essential so that LED LCUs can be used to their full potential and are applied appropriately in any particular clinical situation.
In addition, there is currently no scientific review paper focusing on the LED photopolymerization of available dental materials. This review, therefore, addresses this need and is based mainly on peer-reviewed and frequently cited research articles in international journals available through the Web of Science. This current paper reviews the history of LED photopolymerization in the area of oral biomaterials/dental materials. Within this framework, the basic principles of LEDs, the history and evolution of commercial LED LCUs, the materials science of dental materials photopolymerized with LED LCUs, aspects of commercial LED LCUs as well as their clinical applications are discussed.
2
Basic physics and technology of LEDs
LEDs are a part of our daily lives. LED technology is applied in modern light sources for room lighting, car headlights and dashboards, traffic lights, state-of-the-art television flat screens or as LASER LEDs in CD or blue-ray DVD data/video storage equipment . Compared to conventional light sources, LEDs are small and energy efficient. Hence, dental light curing units (LCUs) based on LEDs are relatively small and can be battery powered, using high performance nickel–metal hydride (NiMH) or lithium-ion (Li-ion) batteries .
Users of dental LCUs are often not fully aware of the physics of these devices. Because light emission of LED LCUs differs greatly from that of other, more traditional types of LCUs, it is worth having a closer look at the physical principles of LEDs. This knowledge may not only help to better understand how LEDs work, but it may also contribute to the appropriate use of LED LCUs in clinical practice and to recognize the strengths and limitations of these devices in daily use.
LEDs are semiconductor-based photonic devices in which the elementary particles of light (photons) play the key role. LEDs convert electrical energy into optical radiation . It has been known for more than one hundred years that light can be generated if an electric current passes through a material under bias . This phenomenon is called electroluminescence and was discovered in 1907 in the natural semiconductor silicon carbide .
LEDs emit light under forward biased conditions. To understand this, one has to consider the energy states of electrons in the semiconductor. Through the quantum mechanical interaction of large numbers of atoms (≅ 10 25 ) and electrons in the solid state, the electronic states may spilt into very closely spaced electron states called electron energy bands . The valence energy band is associated with the highest energy, occupied with electrons at 0 K. The band with the next higher electron energy is called the conduction band and also contains no electrons at 0 K. The valence and conduction bands are separated by a band gap in which the Schrödinger equation has no solution, i.e., no electrons are allowed in this band gap under normal circumstances. For semiconductors, the typical band gap energies are generally less than 2 eV . Fig. 1 shows the band structure of an intrinsic semiconductor.
In intrinsic semiconductors, the electrical behavior is based on the electronic structure inherent in the pure material, such as silicon . If impurity atoms are introduced to dictate the electrical behavior of the semiconductor, it is called an extrinsic (doped) semiconductor. LEDs use both, n-type and p-type doped extrinsic semiconductors, indicating the majority charge carriers are electrons or holes, respectively. Doping a semiconductor with atoms of the group VA of the periodic table creates n-type extrinsic semiconductors, whereas doping with atoms from the group IIIA of the periodic table creates p-type extrinsic semiconductors . The former leads to new, so-called electron donor states in the band gap just below the conduction band, whereas the latter leads to acceptor states in the band gap just above the valence band.
Fifty years ago in 1962, Holonyak and Bevacqua reported the emission of coherent visible light from GaAsP junctions . This event can be considered to be the birth of LEDs emitting visible light . The first visible light LEDs were expensive (approximately 200 USD per unit) and had very few practical applications . The first visible light GaAsP LED emitted red light and had a luminous efficacy of 1.5 lumens per Watt of electrical power consumption (lm W −1 ) . In the early to mid-1980s, these types of LEDs were supplemented by green (GaP:N) and yellow (GaAsP:N) types, respectively.
For materials in which electron and hole conduction exists, the electrical conductivity is strongly dependent on the number of electrons and holes . For practical purposes, only these doped semiconductors are suitable as materials for LEDs. The LED is a diode having p- and n-doped semiconductors that are connected by a junction (p–n-junction). This arrangement ideally allows, under a forward biased condition, an electric current flowing from the p-doped side (anode) to the n-doped side (cathode), whereas no current (ideally infinite resistance) is observed under reverse biased conditions. This effect is based on interactions of the electrical field and the different electron and hole concentrations, respectively, at the p–n-junction .
In doped semiconductor devices, such as LEDs ( Fig. 2 ), electrons and holes (also called defect electrons) contribute to the electric current. Under a forward biased condition (electrons entering the p-doped semiconductor side and exiting through the n-doped layer), electrons and holes recombine near the p–n-junction of the diode and emit photons: an electron in the conduction band can spontaneously return to an empty state in the valence band , during which a photon is produced as well as heat. The frequency of photon emission is controlled by the chemistry of composition of both the p- and n-doped materials.
The width of the band gap is fixed for a particular semiconductor and determines the emitted photon wavelength and, thus, the color of light generated, leading to the characteristic narrow light emission spectrum of LEDs. For example, a relatively wide band gap electron transition results in the emission of blue light, whereas a narrow band gap electron transition results in emission of red light from LEDs. The band gaps in semiconductors can be either direct or indirect. For LEDs, the direct band gap is most important, because this value influences the ability to emit photons through direct conduction band–valence band transitions. This ability means that photon absorption or emission through band transitions is based on the k -selection rule, where k is the crystal-momentum ( k -vector) . The wave vectors of the valence band wave function ( k 1 ) and of the conduction band wave function ( k 2 ) must differ by the amount of the emitted photon wave vector, considering the physical conservation of momentum .
In the early 1990s, the standard LCUs in dental practices and clinics used incandescent QTH light sources in combination with a blue light (band-pass) filter to generate blue visible light. Blue light is necessary to initiate the setting reaction (photopolymerization) in most light cured dental materials. For the realization of the radically new approach of LED LCUs , at least two prerequisites had to be fulfilled. The first prerequisite was the availability of blue LEDs of proper emission wavelength, the second was that blue LEDs needed to have sufficient emitted power to cure dental materials within a reasonable time (20–40 s). Although blue LEDs with a suitable wavelength emission were available from 1971 , their power emission was much too small to be used for clinically relevant exposure times. This situation changed in 1994 when Nakamura at the Nichia Corporation developed high brightness GaN LEDs . From that point in time, the development and scientific exploration of LED photopolymerization of oral biomaterials began.
LEDs have a number of intrinsic advantages which make them ideally suited for the photopolymerization of oral biomaterials. The typical spectral line width of light for LEDs is 5–20 nm . Compared to the emission spectra from all other LCUs, with the exception of narrow band argon-ion lasers, this is extremely narrow ( Fig. 3 ). This narrow emission range is the major advantage of LED LCUs compared to light emitted from LCUs based on different principles, because photo initiators present in oral biomaterials have light absorption spectra with distinct maxima. If the wavelength of the LED LCU is chosen in this range, effective and rapid photopolymerization is the result.
For oral biomaterials containing more than one photo initiator with different light absorption spectra, LED LCUs emitting multiple wavelengths can be employed . These LED LCUs are sometimes called broad band LED LCUs. This term, however, is not correct because the LED emission characteristics (see above) apply. Rather than a broad light spectrum, two or more distinct narrow wavelengths bands are emitted from this collection of different wavelength LEDs, each with a distinct maximum. Thus, these types of LCUs are more appropriately referred to as poly-wave LED LCUs.
Conventional QTH LCUs cannot compete in this respect with LED LCUs because the emission spectrum of the QTH lamp emits a relatively broad visible light spectrum , much of which is useless for photopolymerization and is dissipated as heat. In addition, these lamps require infrared-blocking blue bandpass filters. These filters can deteriorate over time due to the substantial heat the lamp produces.
The second major advantage of LEDs for photopolymerization is their efficiency. The external quantum efficiency is defined as the number of photons emitted externally divided by the number of charge carriers passing through the p–n-junction . Due to the internal properties of the p–n-junction and to the differences in refractive indices at interfaces of the semiconductor and the ambient air, there are losses of photons (i.e., they are not emitted from the semiconductor). Three mechanisms reduce the quantity of photons emitted from the LED: (1) internal absorption, (2) Fresnel loss and (3) critical angle loss . The Lambertian emission pattern, i.e., the angular dependence of light emission is controlled by planar, hemispherical or parabolic epoxy encapsulates on the LED semiconductor .
An important measure of a LED is its luminous efficacy (the ratio of luminous flux emitted to electrical power consumed) and is a measure of how well a light source produces visible light . For LEDs, the luminous efficacy is typically in the order of 60 lm W −1 . LED luminous efficacies of 150 lm W −1 have been attained for white LEDs whereas typical QTH lamps present in LCUs, have a luminous efficacy of only 25 lm W −1 .
When comparing commercially available blue LEDs with conventional QTH lamps in terms of efficiency, the luminous intensity efficiency η is the measure that is most easily obtained from technical data sheets and this is more useful than the external quantum efficiency. The luminous intensity is a photometric measure of the optical radiant intensity which is weighted by the sensitivity of the human eye, with the peak at 555 nm. The luminous intensity I v of a commercial blue LED with an apex angle of 10° which consumes 6.4 mW electrical power is about 9.3 cd . Thus, the luminous intensity efficiency for a LED is η LED = 9.3 cd/6.4 mW = 145 cd W −1 of blue light. A 75 W QTH lamp with the same apex angle of 10° yields 7500 cd . The luminous intensity efficiency of a QTH lamp is, therefore, η QTH = 7500 cd/75 W = 100 cd W −1 across the whole of the visible spectrum.
Another important advantage of LED LCUs is their overall energy efficiency in terms of energy required for a cure cycle. A contemporary LED LCU that has one 5 W LED chip will operate approximately 25 min from a fully charged battery, which may require 10 h at 2.5 W to recharge . Assuming a light curing cycle of 20 s, 75 curing cycles are possible from a charged battery and the energy required for this use is 25 W h −1 , corresponding to 0.34 W h −1 per curing cycle.
In contrast, operating a typical 150 W QTH lamp based LCU, equipped with a 75 W (12 V) QTH source, for the same time (25 min), the energy required would be approximately 62.5 W h −1 . This value corresponds to 0.84 W h −1 per 20 s curing cycle: approximately 2.5 times the energy a LED LCU needs to perform the same task as can be easily calculated.
LEDs can have a typical lifetime of 100,000 h or more and undergo little degradation of light output over this time, if not over-driven . This level of durability is a distinct advantage when compared to the characteristics of QTH lamps which have an effective lifetime of approximately 50 h .
2
Basic physics and technology of LEDs
LEDs are a part of our daily lives. LED technology is applied in modern light sources for room lighting, car headlights and dashboards, traffic lights, state-of-the-art television flat screens or as LASER LEDs in CD or blue-ray DVD data/video storage equipment . Compared to conventional light sources, LEDs are small and energy efficient. Hence, dental light curing units (LCUs) based on LEDs are relatively small and can be battery powered, using high performance nickel–metal hydride (NiMH) or lithium-ion (Li-ion) batteries .
Users of dental LCUs are often not fully aware of the physics of these devices. Because light emission of LED LCUs differs greatly from that of other, more traditional types of LCUs, it is worth having a closer look at the physical principles of LEDs. This knowledge may not only help to better understand how LEDs work, but it may also contribute to the appropriate use of LED LCUs in clinical practice and to recognize the strengths and limitations of these devices in daily use.
LEDs are semiconductor-based photonic devices in which the elementary particles of light (photons) play the key role. LEDs convert electrical energy into optical radiation . It has been known for more than one hundred years that light can be generated if an electric current passes through a material under bias . This phenomenon is called electroluminescence and was discovered in 1907 in the natural semiconductor silicon carbide .
LEDs emit light under forward biased conditions. To understand this, one has to consider the energy states of electrons in the semiconductor. Through the quantum mechanical interaction of large numbers of atoms (≅ 10 25 ) and electrons in the solid state, the electronic states may spilt into very closely spaced electron states called electron energy bands . The valence energy band is associated with the highest energy, occupied with electrons at 0 K. The band with the next higher electron energy is called the conduction band and also contains no electrons at 0 K. The valence and conduction bands are separated by a band gap in which the Schrödinger equation has no solution, i.e., no electrons are allowed in this band gap under normal circumstances. For semiconductors, the typical band gap energies are generally less than 2 eV . Fig. 1 shows the band structure of an intrinsic semiconductor.
In intrinsic semiconductors, the electrical behavior is based on the electronic structure inherent in the pure material, such as silicon . If impurity atoms are introduced to dictate the electrical behavior of the semiconductor, it is called an extrinsic (doped) semiconductor. LEDs use both, n-type and p-type doped extrinsic semiconductors, indicating the majority charge carriers are electrons or holes, respectively. Doping a semiconductor with atoms of the group VA of the periodic table creates n-type extrinsic semiconductors, whereas doping with atoms from the group IIIA of the periodic table creates p-type extrinsic semiconductors . The former leads to new, so-called electron donor states in the band gap just below the conduction band, whereas the latter leads to acceptor states in the band gap just above the valence band.
Fifty years ago in 1962, Holonyak and Bevacqua reported the emission of coherent visible light from GaAsP junctions . This event can be considered to be the birth of LEDs emitting visible light . The first visible light LEDs were expensive (approximately 200 USD per unit) and had very few practical applications . The first visible light GaAsP LED emitted red light and had a luminous efficacy of 1.5 lumens per Watt of electrical power consumption (lm W −1 ) . In the early to mid-1980s, these types of LEDs were supplemented by green (GaP:N) and yellow (GaAsP:N) types, respectively.
For materials in which electron and hole conduction exists, the electrical conductivity is strongly dependent on the number of electrons and holes . For practical purposes, only these doped semiconductors are suitable as materials for LEDs. The LED is a diode having p- and n-doped semiconductors that are connected by a junction (p–n-junction). This arrangement ideally allows, under a forward biased condition, an electric current flowing from the p-doped side (anode) to the n-doped side (cathode), whereas no current (ideally infinite resistance) is observed under reverse biased conditions. This effect is based on interactions of the electrical field and the different electron and hole concentrations, respectively, at the p–n-junction .
In doped semiconductor devices, such as LEDs ( Fig. 2 ), electrons and holes (also called defect electrons) contribute to the electric current. Under a forward biased condition (electrons entering the p-doped semiconductor side and exiting through the n-doped layer), electrons and holes recombine near the p–n-junction of the diode and emit photons: an electron in the conduction band can spontaneously return to an empty state in the valence band , during which a photon is produced as well as heat. The frequency of photon emission is controlled by the chemistry of composition of both the p- and n-doped materials.
The width of the band gap is fixed for a particular semiconductor and determines the emitted photon wavelength and, thus, the color of light generated, leading to the characteristic narrow light emission spectrum of LEDs. For example, a relatively wide band gap electron transition results in the emission of blue light, whereas a narrow band gap electron transition results in emission of red light from LEDs. The band gaps in semiconductors can be either direct or indirect. For LEDs, the direct band gap is most important, because this value influences the ability to emit photons through direct conduction band–valence band transitions. This ability means that photon absorption or emission through band transitions is based on the k -selection rule, where k is the crystal-momentum ( k -vector) . The wave vectors of the valence band wave function ( k 1 ) and of the conduction band wave function ( k 2 ) must differ by the amount of the emitted photon wave vector, considering the physical conservation of momentum .
In the early 1990s, the standard LCUs in dental practices and clinics used incandescent QTH light sources in combination with a blue light (band-pass) filter to generate blue visible light. Blue light is necessary to initiate the setting reaction (photopolymerization) in most light cured dental materials. For the realization of the radically new approach of LED LCUs , at least two prerequisites had to be fulfilled. The first prerequisite was the availability of blue LEDs of proper emission wavelength, the second was that blue LEDs needed to have sufficient emitted power to cure dental materials within a reasonable time (20–40 s). Although blue LEDs with a suitable wavelength emission were available from 1971 , their power emission was much too small to be used for clinically relevant exposure times. This situation changed in 1994 when Nakamura at the Nichia Corporation developed high brightness GaN LEDs . From that point in time, the development and scientific exploration of LED photopolymerization of oral biomaterials began.
LEDs have a number of intrinsic advantages which make them ideally suited for the photopolymerization of oral biomaterials. The typical spectral line width of light for LEDs is 5–20 nm . Compared to the emission spectra from all other LCUs, with the exception of narrow band argon-ion lasers, this is extremely narrow ( Fig. 3 ). This narrow emission range is the major advantage of LED LCUs compared to light emitted from LCUs based on different principles, because photo initiators present in oral biomaterials have light absorption spectra with distinct maxima. If the wavelength of the LED LCU is chosen in this range, effective and rapid photopolymerization is the result.
For oral biomaterials containing more than one photo initiator with different light absorption spectra, LED LCUs emitting multiple wavelengths can be employed . These LED LCUs are sometimes called broad band LED LCUs. This term, however, is not correct because the LED emission characteristics (see above) apply. Rather than a broad light spectrum, two or more distinct narrow wavelengths bands are emitted from this collection of different wavelength LEDs, each with a distinct maximum. Thus, these types of LCUs are more appropriately referred to as poly-wave LED LCUs.
Conventional QTH LCUs cannot compete in this respect with LED LCUs because the emission spectrum of the QTH lamp emits a relatively broad visible light spectrum , much of which is useless for photopolymerization and is dissipated as heat. In addition, these lamps require infrared-blocking blue bandpass filters. These filters can deteriorate over time due to the substantial heat the lamp produces.
The second major advantage of LEDs for photopolymerization is their efficiency. The external quantum efficiency is defined as the number of photons emitted externally divided by the number of charge carriers passing through the p–n-junction . Due to the internal properties of the p–n-junction and to the differences in refractive indices at interfaces of the semiconductor and the ambient air, there are losses of photons (i.e., they are not emitted from the semiconductor). Three mechanisms reduce the quantity of photons emitted from the LED: (1) internal absorption, (2) Fresnel loss and (3) critical angle loss . The Lambertian emission pattern, i.e., the angular dependence of light emission is controlled by planar, hemispherical or parabolic epoxy encapsulates on the LED semiconductor .
An important measure of a LED is its luminous efficacy (the ratio of luminous flux emitted to electrical power consumed) and is a measure of how well a light source produces visible light . For LEDs, the luminous efficacy is typically in the order of 60 lm W −1 . LED luminous efficacies of 150 lm W −1 have been attained for white LEDs whereas typical QTH lamps present in LCUs, have a luminous efficacy of only 25 lm W −1 .
When comparing commercially available blue LEDs with conventional QTH lamps in terms of efficiency, the luminous intensity efficiency η is the measure that is most easily obtained from technical data sheets and this is more useful than the external quantum efficiency. The luminous intensity is a photometric measure of the optical radiant intensity which is weighted by the sensitivity of the human eye, with the peak at 555 nm. The luminous intensity I v of a commercial blue LED with an apex angle of 10° which consumes 6.4 mW electrical power is about 9.3 cd . Thus, the luminous intensity efficiency for a LED is η LED = 9.3 cd/6.4 mW = 145 cd W −1 of blue light. A 75 W QTH lamp with the same apex angle of 10° yields 7500 cd . The luminous intensity efficiency of a QTH lamp is, therefore, η QTH = 7500 cd/75 W = 100 cd W −1 across the whole of the visible spectrum.
Another important advantage of LED LCUs is their overall energy efficiency in terms of energy required for a cure cycle. A contemporary LED LCU that has one 5 W LED chip will operate approximately 25 min from a fully charged battery, which may require 10 h at 2.5 W to recharge . Assuming a light curing cycle of 20 s, 75 curing cycles are possible from a charged battery and the energy required for this use is 25 W h −1 , corresponding to 0.34 W h −1 per curing cycle.
In contrast, operating a typical 150 W QTH lamp based LCU, equipped with a 75 W (12 V) QTH source, for the same time (25 min), the energy required would be approximately 62.5 W h −1 . This value corresponds to 0.84 W h −1 per 20 s curing cycle: approximately 2.5 times the energy a LED LCU needs to perform the same task as can be easily calculated.
LEDs can have a typical lifetime of 100,000 h or more and undergo little degradation of light output over this time, if not over-driven . This level of durability is a distinct advantage when compared to the characteristics of QTH lamps which have an effective lifetime of approximately 50 h .
3
Materials science of dental materials photopolymerized with LED LCUs
There are several well-known examples in the history of science that show that change and new ideas are not always welcomed enthusiastically and can be even opposed. This situation can be particularly the case if this change happens quickly and has the character of a paradigm shift. To some extent, this circumstance was also the case with the introduction of LED LCUs to photopolymerization of oral biomaterials. The authors recall submitting one of their first scientific papers reporting the novel concept of LEDs used for the photopolymerization of oral biomaterials to a renowned international journal. This manuscript was rejected, based on a referee’s comment essentially stating that one cannot cure dental materials with LEDs.
In the early 1990s, the most common LCUs in dentistry were QTH units. Degradation of the QTH lamp, filter aging, contamination of the light-guide tips, and several other factors, potentially reduced the light output of these devices over time. Nevertheless, users of LCUs did generally not monitor the light output of these devices over time and the radiometers needed to do so were not available in most dental practices.
Against this background, in 1995 Mills wrote a letter to the British Dental Journal wondering if LEDs could be used as an alternative light source for the photopolymerization of dental composites . Shortly before this letter was published, the Nichia Corporation in Japan introduced high intensity blue GaN LEDs having an emission peak around 465 nm. The former letter mentioned further a simple photopolymerization test of one composite sample .
The first scientific peer-reviewed article published in an international journal presenting data on photopolymerization of three contemporary dental composites with a LED LCUs was published a few years later . In this paper, the hypothesis was tested, that a blue LED LCU can produce an equal composite depth of cure as a QTH LCU adjusted to give the minimum effective irradiance accepted at that time: 300 mW cm −2 . This paper is a milestone in the history of LED photopolymerization and to date, is the most cited article on LED photopolymerization of dental composites . The reasons for this acknowledgment are threefold. First, the article presented a new technological approach for photopolymerization of oral biomaterials and for the first time a new powerful LED LCU was introduced in a peer reviewed scientific article . At that time, single blue LED emitters were not strong enough to emit enough light to polymerize dental composites satisfactorily and within a reasonable time. Therefore, this first LED LCU used 25 blue LEDs in an array. Second, the paper presented the first graph showing simultaneously, the spectral flux and spectral irradiance of a conventional QTH LCU and a LED array based LCU. In addition, it was also shown that the light emitted from the LED LCU correlated well with the peak of the absorption of the camphorquinone photoinitiator. Much of the light emitted by the QTH LCU, however, was not effective for photopolymerization in composites using the camphorquinone photoinitiator. Third, the paper demonstrated that a LED LCU with an irradiance of 290 mW cm −2 was able to cure three popular commercial dental composites to statistically significantly greater depths of cure than was a QTH LCU which had a 60% greater irradiance of 455 mW cm −2 . Although the light emission characteristics of QTH LCUs and the light absorption characteristics of the photoinitiator camphorquinone were already known, this article again raised awareness of the characteristics of the light emitted from different LCUs and their effects on the properties of the photopolymerized materials. In addition, this work triggered a new dynamic of research in the area as is evident from the high number of citations of this paper in subsequent published research.
In a further study, the depths of cure and compressive strengths of a dental composite of two different shades, each polymerized with a QTH LCU or a LED LCU, respectively, were compared . The QTH LCU had an irradiance of 755 mW cm −2 whereas the LED LCU delivered 350 mW cm −2 emitted from 27 blue LEDs ( Fig. 4 ). In addition, an advanced characterization of the light of both LCUs was performed. Both LCUs were able to produce a depth of cure exceeding the requirements of ISO 4049 and the compressive strength of the composites did not statistically significantly differ between the LCUs, despite that fact the LED LCU had less than half the irradiance of the QTH LCU. This result was explained by the light emission spectrum of the LED LCU providing more radiant energy within the peak spectral range of camphorquinone than that measured from the QTH device. A later study confirmed that camphorquinone has practically the highest photopolymerization efficiency when irradiated with blue LEDs compared to other photoinitiators, despite its rather low polymerization quantum yield . This finding showed clearly that LED LCUs were more effective in the photopolymerization of dental composites than were QTH lights.
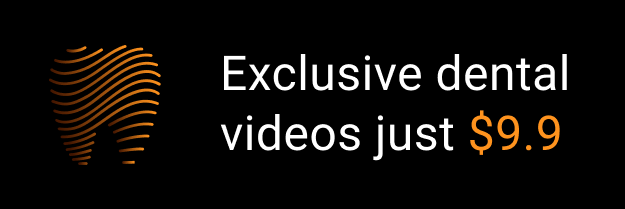