Bone Response to Mechanical Loads
Girish Ramaswamy, Martha Warren Bidez, Carl E. Misch
In an effort to optimize the interaction between bone and dental or skeletal implants, many researchers have focused their attention on the implant-tissue interface.1–19 The importance of the events that take place at the interfacial “zone” between an implant and the host tissue cannot be overstated. This complex interaction involves not only biomaterial and biocompatibility issues but also the alteration of the mechanical environment that occurs when placement of an implant disturbs the normal physiologic distribution of forces, fluids, and cell communication. The purpose of this chapter is to describe the current state of understanding regarding the biological and biomechanical response of bone to mechanical loads, with particular emphasis on the dental implant–bone interface.
Biological Response
The implant-tissue interface is an extremely dynamic region of interaction. This interface completely changes character as it goes from its genesis (placement of the implant into the prepared bony site) to its maturity (healed condition). The biomechanical environment plays an immediate role in the quality and compositional outcome of the new interface. For example, extensive research shows that if the implant is stable in the bone at the time of placement, then the interface is more likely to result in osteointegration.15,20 Relative movement (or micromotion) between the implant and the bone at the time of placement is more likely to favor the development of a fibro-osseous interface.11,18 The healing stage of the interface, however, is only the beginning of its dynamic nature. Functional loading of the implant brings additional biomechanical influences that greatly affect the composition of this junction.
A topic of intense research for many years is the transduction of loading-induced strain at the interface into a signal that can direct the interfacial tissues to respond or remodel. It has been proven that bone responds to both hormonal and biomechanical (functional loading) regulation. These two regulating mechanisms are often in opposition to each other. Research has shown that even in instances in which a large demand exists for calcium (the primary objective for hormonal regulation), functional loading can compete and maintain bone mass.21 Researchers have theorized that the actual strain (see Chapter 22) that is perceived by the bone tissue initiates a chain of events that results in a biological response. For tissue strain to influence bone adaptation at the bone-implant interface, it must elicit some sort of a chemical or biological response in a strain-sensitive population. The current hypothesis is that bone cells in conjunction with the extracellular matrix (ECM) comprise the strain-sensitive population and that each plays a vital role in the mediation of the interface. Based on this rationale, the objective of a good implant design would be to establish and maintain a strain environment within the host bone tissue and at the interface that favors osteointegration of the implant.
Mechanotransduction
Mechanotransduction is a multistep process that includes (1) mechanocoupling (transduction of mechanical forces into signals sensed by sensor cells), (2) biochemical coupling (conversion of mechanical signal into a biochemical signal to elicit a cellular response such as gene activation), (3) transfer of a signal from sensor to effector cells, and (4) the effector cell response.22 Recent studies have led to the current consensus that osteocytes embedded within lacunae in bone matrix act as mechanosensors and help translate mechanical loads into biochemical signals.23–25 Osteocytes are the most abundant cells and inhabit an extensive lacunocanalicular network that enables them to communicate with other osteocytes, as well as with periosteal and endosteal osteoblasts.24–26 Furthermore, osteocytes have higher sensitivity to mechanical stimulation than osteoblasts.27–29
The strains experienced at the tissue level in vivo during normal activities (0.04%–0.3%) are much less than the strain levels (1%–10%) required to elicit a cellular response.30,31 Initially, shear stress caused by fluid flow in the canalicular spaces was believed to be the primary driver of biochemical coupling in bone, with strain being the dominant stimulus.23,32 Later, You et al.33 proposed a model for the amplification of physiologically induced strains to levels that would initiate intracellular biochemical responses. The model suggested that drag forces on the transverse tethering fibers34,35 during fluid flow through the pericellular space (filled with matrix between osteocyte cell membrane and canalicular wall) will produce a tensile stress, which will in turn create a hoop strain in the intracellular actin cytoskeleton that is two orders of magnitude higher than the strains at the whole-bone level. This model was further modified using updated ultrastructural data on the cytoskeleton, transverse tethering fibers, and their structural rigidity.36 Cowin37 compared and reviewed the two models by focusing on the relationship between the bone microstructure and the mechanism by which osteocytes sense the fluid flow as the result of mechanical loading. Possible mechanoreceptors, mechanisms by which osteocytes sense mechanical stimuli, and intracellular signaling pathways are discussed in several research groups.38–40 Regulation of osteoblastic activity by osteocytes has been proposed to occur through gap junctions,41–44 with the stimulation of gap junctions mediated by prostaglandin E2 (PGE2).45–49
Whereas loading of bone decreases osteocyte apoptosis, disuse and supraphysiologic strains increases it followed by haversian remodeling.50–52 Bone disuse, even for short durations, may rapidly induce a hypoxic state of stress in osteocytes, which when extended may lead to apoptosis. This hypoxia can be reversed by short-term physiologic loading, which suggests that mechanical loading at such magnitudes plays a key role in osteocyte viability.53 This may adversely affect bone strength independent of bone loss.54 Hypoxic osteocytes may also mediate disuse-induced bone resorption by increasing osteopontin expression.55
Biomechanically Based Bone-Remodeling Theories
Biomechanically based bone-remodeling theories largely fostered the desire to optimize the effects of strain at the bone-implant interface to encourage osteointegration. In 1887, Meier56 described the systematic structure of trabecular bone in the femoral head. In 1892, Wolff57 described these events as a law of nature and stated that the trabecular bone will place or displace itself in relationship to the functional pressures. In 1895, Roux58 suggested that the tissue changes to loading were a result of a cellular-regulation process. Frost59 proposed the theory of the mechanostat. He postulated that bone mass is a direct result of the mechanical use of the skeleton. This agrees with Wolff’s law57 that essentially states “form follows function.” Frost established a mechanical-adaptation chart relating trivial loading, physiologic loading, overloading, and pathologic loading zones to ranges of microstrain (Figure 6-1). His studies showed that strains in the range of 50 to 1500 microstrain (mε) stimulated increases in cortical bone mass until the strains were reduced to the threshold range (or minimum effective strain). This process of the mechanostat would effectively switch the bone modeling on and off. This phenomenon led him to the flexure drift hypothesis in which he proposed that long bones (e.g., femur) were geometrically curved to minimize the strain distribution down the long axis of the bone.60 Frost suggested that the curvature of the long bones canceled the bending moment caused by the eccentric pull of the muscles.
Bone may reduce strains by bone apposition or reduction, by bone formation or resorption, and by changing modulus of elasticity or stiffness by changing mineral content.61–63 Necrosis of bone cells appears to determine the upper equilibrium level. Whereas cell destruction can be observed when stresses exceed 6.9 µ 10 N/mm2, a stress of 2.48 µ 10 N/mm2 will cause an increase in bone growth.64
Turner et al.23 and Turner65 summarized the rules governing bone adaptation as (1) dynamic (not static) loading drives bone adaptation; (2) whereas short-term loading has an anabolic effect, increased duration degrades bone adaptation; and (3) whereas abnormal strains evoke bone adaptation, bone becomes accustomed to routine strains and remodeling ceases.
Dynamic loading has consistently been found to have more osteogenic potential than static loading.66 Dynamic axial loading for short durations, which produced strains within the physiological range that were added to normal activity, led to adaptive straightening of growing rat ulnae. Reduced and increased periosteal bone formation were observed at moderate and higher peak strains, respectively.67 Similar studies observed adaptive osteogenic response to be proportional to the strain rate and local surface strain.68,69
Contrary to the previously stated anabolic responses by rat ulna, both static and dynamic axial loading have been found to cause a reduction in longitudinal bone growth.70,71 Growing male rats70 receiving 10-minute bouts of static loading at 17 N, static loading at 8.5 N, or dynamic loading at 17 N exhibited shorter bone growth than the control subjects. The suppression was mainly visible in the hypertrophic zone and was proportional to the load magnitude. A later study71 investigated the growth plate biology after the application of three different compressive loads (4 N, 8.5 N, and 17 N) for 10 min/day for 8 days on rat ulnae. The longitudinal mineralization rate was completely suppressed and never recovered in the 17-N rats, but other groups showed significant suppression that recovered within 1 week after loading. From their results, the authors suggested that even low-magnitude compressive loads suppress growth rate (Figure 6-2), which supported Hert’s proposal72 and deviates from Frost’s chondral growth force response curve.73
Studies show that adaptive osteogenic response attains saturation after the initial few cycles during continuous cyclic loading despite further increases in magnitude of load and cycle number.74 Dynamic loading in short bouts (i.e., with rest periods inserted between loading) have been found to induce an increase in the number and activity of osteoblasts75,76 and enhance osteogenesis in normal and aged skeletons76–79 during normal activities such as walking. This consequently improves the biomechanical integrity of the bone despite only slight increments in bone mineral density and content.80 Rest-inserted loading decreased the threshold for lamellar bone formation,81 reduced the number of cycles required to stimulate bone formation, and promoted an increased osteogenic response at any load magnitude (Figure 6-3).82
Recently, Gross et al.82 hypothesized that rest periods between each cycle in cyclic loading enhances fluid flow through the canalicular network, thereby extending the communication range of osteocytes by improving transport of signaling molecules between them. Furthermore, rest-inserted loading may also turn on synchronized activity among osteocytes.
Osteogenic response has been found to vary with respect to anatomical site and even at different regions within the same bone. Axial compressive dynamic loading of adult female rat ulnae led to greater periosteal lamellar bone formation distally and lower bone formation proximally compared with the middiaphysis. Strain thresholds and periosteal lamellar bone formation correlated with the peak strains experienced in rat ulnae being higher distally than proximally with intermediate values at the diaphysis.83 Results from a previous study showed that treadmill exercise increased cancellous bone at the distal tibia more markedly than the proximal tibia, but vertebra showed no change.84 A more recent study in mice revealed that the metaphyseal region in the distal femur showed an increased osteogenic response compared with the cortical bone at the middiaphysis.85 Loading of the knee increased bone formation and mineral apposition on the medial side of the tibial diaphysis compared with the lateral and posterior sides.86
Small increases in such mechanical signals to which bones are exposed during regular activities such as standing produce a local, rather than systemic, adaptive anabolic response in cancellous bone without any effect on cortical bone.87,88 Anabolic effects on trabecular bone were attributed to an increase in bone mineral content and trabecular number, but decreases in trabecular spacing indicated the creation of new trabeculae and thickening of existing ones. In addition, researchers observed the adaptation of trabecular bone from rod shaped to plate shaped, primarily in the weight-bearing direction, consequently exhibiting increased strength and stiffness when measured longitudinally.89 Short bursts of such stimuli inhibit bone resorption by reducing osteoclastic activity and concurrently increase bone formation, maintaining the bone matrix properties.90 This anabolic response is not controlled by matrix strain magnitude but by applied frequency.91 Controlled compressive load producing a peak pressure of 1 MPa was applied for 10, 25, or 50 cycles/day at a frequency of 0.5 Hz for 1 month on the distal femoral condyles of rabbits. The loaded limbs showed increased bone volume fraction, trabecular thickness, mean intercept length, and mineral apposition rate compared with unloaded contralateral limbs.92 Such low-magnitude, high-frequency mechanical signals (whole-body vibrations) have high potential in the treatment of osteoporosis.93
Mechanical stimulation has also been used to accelerate bone formation during fracture and trauma. Whereas in vivo dynamic axial compression loading of low magnitude after a short delay increased the strength of fracture callus, immediate loading and shear movement inhibited and delayed the healing process.94,95 Alternate axial compression and distraction, called dynamization, was found to be more effective than either technique applied alone; this combination stimulated callus at both central (influenced by distraction) and peripheral (influenced by compression) regions in a closed transverse fracture in rat tibiae.96
Indicators of the Biological Response
The characterization of the biological responses resulting from cellular deformation is equally as diverse as the deformation methodologies. These include changes in the concentration of intracellular mediators and cellular proliferation.
Changes in Concentration of Intracellular Mediators
Numerous investigators have reported fluctuation in the concentrations of intracellular second-messenger molecules.97–112 In general, cell surface receptors relay information by activating a chain of events that alters the concentration of one or more small intracellular-signaling molecules often referred to as second messengers or intracellular mediators. In turn, these messenger molecules pass the signal on by altering the behavior of selected cellular proteins. Some of the most widely used intracellular mediators are cyclic AMP (cAMP), Ca2+, and cyclic GMP (cGMP).113 PGE2 and prostacyclin are paracrines that are released by osteoblasts in response to mechanical strain.97,104–108 They are essential for bone formation by mechanical loading114–119 and are also increased by fluid shear stress120–125 in a dose-dependent manner. The anabolic effect of mechanical stimulation in vivo has been shown to be greatly depressed by the addition of indomethacin, a chemical that blocks the production of these prostaglandins (PGs).109 Increase in messenger ribonucleic acid (mRNA) of c-fos and insulin-like growth factor 1 (IGF-1) in osteocytes immediately after mechanical stimulation led to the study involving compressive dynamic loading of the eighth caudal vertebrae in rats. When indomethacin and NG-monomethyllarginine (L-NMMA), inhibitors of PG and nitric oxide (NO), respectively, were administered individually, c-fos (a marker for mechanical responsiveness in osteocytes) was suppressed partially, but combined administration resulted in drastic suppression.126
This suggested that PG might be produced by NO-dependent and NO-independent mechanisms. Rats injected with NO donors showed increased osteogenic response only when loaded, which suggested that NO requires other molecules such as PG induced by mechanical loading for bone.117 Human bone cells from patients with osteoporosis subjected to pulsating fluid flow showed reduced long-term release of PGE2, suggesting that long-term adaptive response of these bone cells to mechanical stimuli may have been affected.127
Harrell and Binderman99 observed that isolated osteoblasts, grown on a polystyrene plate that had an orthodontic jackscrew glued to its bottom, responded to continuous strain by increasing PGE2 concentrations followed in minutes by an increase in cAMP release. Rodan et al.100 agreed that mechanical strain affected the second-messenger cAMP and also reported changes in cGMP and calcium ions. Yeh and Rodan97 suggested that PGs might be involved in the transduction of mechanical strain but did not apply physiological levels of strain to their samples. Fluid shear experiments by Reich and Frangos101 and cyclic biaxial strain studies by Brighton et al.98 have demonstrated that osteoblasts respond with an increase in cellular levels of inositol triphosphate.
Osteoblasts form bone by secreting many extracellular matrix proteins, including type I collagen, osteopontin, osteocalcin, osteonectin, biglycan, and decorin. Osteopontin was first purified from rat bone matrix and is considered to play an important role in the cascade of events required for the formation of bone matrix.110 In vitro studies have revealed osteoblasts to be more responsive to fluid forces than mechanical strain, associating increased osteopontin expression with increases in force magnitude without any dependence on strain magnitude or rate.128 Recently, experiments on the femoral epiphyses of rabbits have shown that cyclic loading can influence endochondral bone formation by accelerating formation of secondary ossification centers and increase expression of RUNX2 (an important transcription factor of osteoblasts) and extracellular proteins, including osteopontin, type X collagen, and decorin.129 Osteocalcin, also known as bone Gla protein, is widely used as a marker for bone metabolism. Studies have shown that the production of osteocalcin can be stimulated by mechanical stress both in vivo112 and in vitro.113 Experiments on bone marrow stromal cells have revealed that shear caused by fluid flow enhances maturation of osteoblasts by stimulating the expression of osteocalcin,130 osteopontin, and bone sialoprotein131 but not proliferation of stromal cells.
Parathyroid hormone (PTH) has been found to play a vital role in bone adaptation to mechanical stimuli. Rats with thyroparathyroidectomy did not show any osteogenic response caused by mechanical loading of vertebrae,132 but the response could be restored by a single PTH injection before loading. However, the restoration did not occur when PTH was injected 3 days after mechanical stimulation. Expression of c-fos was observed only in loaded rats injected with PTH,132 further highlighting the importance of PTH in mechanical adaptation of bone. In vitro studies on mouse osteoblasts provided further insights into the interactive effects of PTH and pulsating fluid flow on PGE2 and NO production. Although fluid flow stimulated a twofold rise in PGE2 and NO production, PTH induced a similar effect on PGE2 but reduced NO production by degrading the enzyme activity of NO synthase. When applied together, the stimulatory effects of fluid flow were nullified. According to the authors, the results suggested that PTH enhances NO-independent PGE2 production but inhibits stress-induced NO production by degrading NO synthase, in turn reducing NO-dependent PGE2 production.133 PTH may also regulate mechanotransduction by influencing the influx of extracellular calcium in hypotonic osteocytes.134
Changes in Cellular Proliferation
As previously discussed, the response of osteoblast-like cells to mechanical strain has been shown to be variable. Many studies have reported increases in cell proliferation,98,102,135–137 total protein production, and DNA synthesis135,136,138,139 in response to mechanical strain. A review by Burger and Veldhuijzen31 suggested that at high magnitudes of strain, osteoblasts proliferate and decrease their production of osteoblast phenotypic markers, such as alkaline phosphatase and bone matrix proteins. At lower magnitudes of strain, osteoblasts exhibit a more differentiated state, with an increase in alkaline phosphatase and matrix protein production and a decrease in proliferation. Strains of physiological magnitude (1000 µε) applied by cyclic dynamic stretching on human osteoblast cultures increased cell proliferation and osteoblast activities related to matrix production but decreased alkaline phosphatase and osteocalcin release.140–142 The frequency and cycle number affect proliferation of bone cells and expression of various osteoblast genes in a different manner.143,144 Applying uniaxial strain at a constant frequency, the cell number increased up to 1800 cycles. At a constant cycle rate, frequency variation produced only slight differences. Frequencies of 1 Hz and 300 cycles were optimum, having the maximum positive effect of cell proliferation.144
In addition to experiments correlating increased proliferation with increased or altered strain levels, several investigators have focused their attention on the timing of the proliferative response. Studies conducted by Lanyon145 have shown that cellular metabolism is activated within the first few minutes of loading. Raab-Cullen et al.146 investigated the pattern of gene expression in the tibial periosteum shortly after in vivo controlled external load application. They documented that mRNA expression was altered within 2 hours after loading and that the pattern of specific mRNA expression first reflected proliferation and subsequently differentiation. Cyclic equibiaxial stretching147 of 7-day osteoblast cultures increased apoptosis independent of the strain range (0.4%–2.5%), but more mature cell culture (2 weeks) increased proliferation. This study revealed the importance of the differentiation stage of osteoblasts in their response to mechanical stimulation.
Chondrogenesis at the periosteum of long bones possesses both osteogenic and chondrogenic potential and holds clinical significance in the repair of articular cartilage and in fracture healing.148–151 Dynamic fluid pressure was found to increase proliferation of periosteal chondrocytes from immature rabbits in vitro.152 The possible chondrogenic effects stimulated by continuous passive motion of joints after periosteal arthroplasty via dynamic fluid pressure were investigated using periosteal explants suspended in agarose gel. Whereas low-level pressure application increased chondrogenesis and type II collagen in a dose-dependent manner, high-pressure completely inhibited these activities.153
Changes in Cellular Morphology and Organization
Ives et al.,154 using human and bovine endothelial cells, found that the cells responded differently to various types of strain. The cells oriented themselves parallel to the direction of shear strain induced by fluid flow but perpendicular to the axis of mechanical deformation on a cyclically stretched polyurethane membrane. Investigations by Buckley et al.,136 using osteoblast-like cells stimulated by cyclic mechanical strain, also resulted in the alignment of the cells perpendicular to the strain vector. This perpendicular alignment was noted at 4 hours after loading and was significant by 12 hours. They suggested that the preferred orientation might have resulted from a mechanical effect on the osteoblast, wherein cell attachments were broken in the maximum strain direction, leaving only those attachments already present in the least strained conformation. A second hypothesis suggested that the cells may have resolved their focal contacts and migrated in an attempt to minimize the strain to which they were subjected.
Another study involving osteoblast-like cells was reported by Carvalho et al.155 They investigated cytoskeletal organization in mechanically strained alveolar bone cells isolated from the alveolar processes of Sprague-Dawley rats. The earliest change in cytoskeletal organization was noted at 30 minutes after the initiation of strain. They observed that the cells oriented themselves perpendicular to the long axis of the applied mechanical strain.
In vitro studies on osteoblastic and osteocytic cell lines subjected to unidirectional and oscillatory fluid shear stresses showed that stress fibers formed and aligned in osteoblasts within 1 hour of unidirectional stress but were delayed in the latter type of stress. Osteocytes show alignment for unidirectional stress and dendritic morphology for oscillatory stress only after 24 hours.156
Altered Expression and Reorganization of Osteoblast Integrins
Although changes in the distribution of the cytoskeleton in mechanically strained cells have been reported, the exact mechanism for the initial detection and transduction of mechanical force into a biological signal has yet to be determined. One possible transduction pathway is the ECM integrin cytoskeletal axis.22,155,157 To understand how the cells interact with the ECM, attention must be given to the nature of the attachment.
Integrins are the primary receptors used by animal cells to attach to the ECM,158 and they function as transmembrane linkers that mediate bidirectional interactions between the ECM and the actin cytoskeleton. Integrins are composed of two noncovalently associated transmembrane glycoprotein subunits called α and β, both of which contribute to the binding of the matrix protein. Electron micrographs of isolated integrins suggest that the molecule has approximately the shape shown in Figure 6-4, with the globular head projecting more than 20 nm from the lipid bilayer. After the binding of a typical integrin to its ligand in the matrix, the cytoplasmic tail of the β chain binds to both talin and α-actinin and thereby initiates the assembly of a complex of intracellular attachment proteins that link the integrin to actin filaments in the cell cortex37,158 (see Figure 6-4). This process is thought to be how local contacts form between cells and the ECM. If the cytoplasmic tail of the β chain is removed or mutated using recombinant DNA techniques, then the integrins can still bind to the matrix, but the strength of the bond is decreased, and the integrins no longer cluster at the focal contacts.158
The connection between integrins and the actin cytoskeleton is considered to be a possible pathway for sensing mechanical signals and producing a response in bone.159–161 The interactions that integrins mediate between the ECM and the cytoskeleton play an important part in regulating the shape, orientation, and movement of the cells.162 Schwartz and Ingber163 suggested a direct link between mechanical strain and cellular response. Integrins of endothelial cells subjected to shear stress were shown to realign with the direction of flow, suggesting that cell adhesion is a dynamic process responding to mechanical strain.164 Wang et al.165 demonstrated that a physical strain applied directly to integrins using a magnetic twisting device was shown to be resisted by the cytoskeleton. Pavalko et al.161 performed in vitro studies on MC3T3-E1 osteoblasts to analyze the role of actin and actin–membrane interactions in altering gene expression because of mechanical loading. Observations of reorganization of actin filaments into contractile stress fibers, formation of focal adhesions, and recruitment of β1-integrins and α-actinin to focal adhesions revealed a critical role played by actin cytoskeleton in altering gene expression (upregulation of cyclooxygenase-2 and c-fos) in osteoblasts as a response to fluid shear stress. Increase in the number and size of stress fibers and focal adhesion complexes associated with mechanical strain indicated a combined change in both cytoskeleton and ECM favoring tighter adhesion of osteoblasts to the latter.160 Both cell adhesion and mechanical stimulation induce expression of integrin-binding proteins—osteopontin, fibronectin, and bone sialoprotein—by osteoblasts but via different mechanisms at different time frames after stimulation. Although strain-induced (dynamics biaxial strain of 1.3% at 0.25 Hz) osteopontin expression was dependent on cytoskeletal integrity, cell adhesion was not.166–168 These observations indicate that the ECM integrin cytoskeletal system may be part of the cascade responsible for the transduction of mechanical strain into a biological response.
Other studies have shown that several intracellular signaling pathways are activated coincident with a clustering of integrins at the focal contacts between the cells and the matrix. These clustered integrins may generate intracellular signals by initiating the assembly of a signaling complex just inside the plasma membrane, similar to that of growth factor receptors. Many cells in culture will not respond to growth factors unless the cells are attached via integrins to the ECM molecules.158,169 Recent investigations have related the extracellular signal-regulated kinase (ERK) pathway (one of the mitogen-activated protein [MAP] kinases identified) to growth and differentiation of osteoblasts,170 differentiation of mesenchymal stem cells toward osteogenic lineage,171 and mechanotransduction.172–179 Whereas fluid flow applying physiological strain levels on human osteoblasts rapidly induced ERK phosphorylation and clustering of αvβ3 integrins in vitro,176 the mechanism behind the regulation of osteocyte apoptosis by mechanical stimulation involves and requires the activation of an integrin–cytoskeleton–Src–ERK pathway.179 These results have led researchers to suggest that both mechanical (e.g., fluid flow, cyclic stretching) and chemical (e.g., hormones, growth factors) stimuli may act through the same intracellular signaling pathways.176,179
Numerous subunits have been characterized, and different combinations of α and β subunits function as receptors for a variety of extracellular proteins.180,181 The β1-integrin subunit is often expressed in bone cells both in vitro and in vivo.181 Carvalho et al.155 demonstrated that changes in the organization of the β1-subunit were induced by the application of strain as early as 4 hours from its onset. They compared the expression of the β1-integrin subunit mRNA from strained cultures with unstrained controls.
Changes in Gene Expression
To characterize the biological response of osteoblast-like cells to external mechanical loading, many researchers are investigating strain-induced alterations in patterns of osteoblast gene expression. Several authors have reported that the initial response to strain is a rapid increase in c-fos mRNA expression, indicative of increased proliferation, paired with a rapid decline in levels of mRNA encoding bone matrix proteins, such as type I collagen, osteopontin, and osteocalcin.146,182 A “rebound” effect or reversal of this trend is usually seen with time as the proliferation tapers off, accompanied by an increase in expression of the matrix proteins.146,182–184
The term matrix proteins refers to both collagenous and noncollagenous proteins. Type I collagen is the most abundant protein in the organic matrix of bone. This molecule is composed of one α2 and two α1 chains. These three chains are initially assembled into a triple helical structure within the cell and are subsequently bundled into fibrils once secreted from the cell. These extracellular fibrils are arranged in a specific, repeating orientation that produces the typical banded appearance common to type I collagen. Intermolecular cross-links stabilize this pattern and produce a porous, repeating, three-dimensional structure.21 Active osteogenesis involves the expression of genes that result in the production of collagen type I protein.184 This trait makes the type I collagen molecule a valuable indicator of differentiated osteoblastic activity. Cyclic pressure increases mRNA expression for type 1 collagen and accumulation of calcium by improving osteoblast function without affecting the cell number.185 Cyclic stretching of rat calvarial osteoblasts increased collagen production at lower strains (500 µε) and inhibited production at higher strain levels (1500 µε).186
In the past 20 years, noncollagenous proteins have received increased attention. Researchers have suggested that these minor components of organic bone matrix may play a role in regulating bone function, expression, and turnover.
Osteocalcin (bone Gla protein) is a noncollagenous protein that binds calcium and has been isolated from bone, dentin, and other mineralized tissues. It is specifically synthesized by differentiated osteoblasts and, similar to type I collagen, is an ideal marker for osteoblast phenotypic expression.187
Another noncollagenous protein that is generating great interest is osteopontin. This bone sialoprotein is synthesized by primary osteoblasts and has been shown to play a role in cell attachment and spreading. Osteopontin contains a binding sequence that appears to be recognized by an integrin cell surface receptor related to the vitronectin receptor.187
Both osteocalcin and osteopontin are regulated by a number of hormones and growth factors. The most common promoter of osteocalcin and osteopontin expression and secretion is 1,25-dihydroxyvitamin D3 (1,25[OH]2D3), which directly influences the genes of both proteins. This is possible because the genes for both osteopontin and osteocalcin contain regions that recognize vitamin D.183 A study by Harter et al.183 analyzed the expression and production of bone matrix proteins in human osteoblast-like osteosarcoma cells in response to 1 to 4 days of chronic, intermittent, mechanical strain. Northern analysis for type I collagen detected an increase in collagen message after 48 hours of strain. Immunofluorescent labeling of type I collagen indicated that secretion was also enhanced. In the absence of vitamin D, osteopontin message levels were increased severalfold by the application of mechanical load. This increase in osteopontin expression was doubled when the cells were subjected to mechanical load in the presence of vitamin D.
Osteocalcin secretion was also increased with cyclic strain. Osteocalcin levels were not detectable in vitamin D–untreated control cells; however, after 4 days of induced load, significant levels of osteocalcin were observed in the medium. With vitamin D present, osteocalcin levels were four times higher in the medium of strained cells compared with unstrained controls. This study demonstrates that mechanical strain of osteoblast-like cells is sufficient to increase the transcription and secretion of matrix proteins via mechanotransduction without hormonal induction.183 Osteoblasts in mechanically loaded mouse periodontium showed increased expressions of osteocalcin, type I collagen, and alkaline phosphatase.188 The first two were more responsive and were found to be stimulated within a short time after loading. The authors suggested that mechanical stimulation drives rapid differentiation of committed osteoblast precursors to produce an anabolic skeletal adaptation.189 Weight loading of chicks at the prepubertal stage led to development of shorter bones with narrower growth plates but with increased mineralization and vascular penetration. Increased osteopontin and matrix metalloproteinases (MMP9 and MMP13) led to the speculation by the authors that MMPs allowed greater penetration of blood vessels carrying osteoblasts and osteoclasts, but osteopontin increased osteoclast numbers, thereby increasing resorption at the growth plate region.190
Disruption of genes can alter the normal bone formation response to mechanical stimulation as observed in mice lacking thrombospondin 2191 that showed contrasting behavior compared with wild-type mice with increase in endocortical bone formation despite higher strains at the periosteal surface.
Frost59 has reported that the mechanism for the biomechanical response of osteoblasts is not discrete. Osteoblastic products such as interleukin-1 (IL-1) can stimulate osteoblasts. He groups these cells as basic multicellular units (BMUs). These BMUs are most prevalent on periosteal and endosteal surfaces, and the periosteal BMUs are most sensitive to biomechanical stimuli.
Limitations of Previous Studies
Although these cell culture studies generate promise for the quantitative delineation of the mechanically induced cellular response of bone, the enthusiasm for all of these studies must be tempered in light of the experimental models that were used. Virtually all of these models used some form of polyurethane membrane, collagen ribbon, or silastic plate as the substrate on which the cells were grown and mechanically stimulated. Given the complex host-biomaterial interactions within the human body, the cellular response of isolated bone cells on polyurethane membranes or collagen ribbons may be significantly different from bone cells in intimate contact with a contemporary implant biomaterial, such as titanium or titanium alloy. Investigations are in progress to confirm the effects of mechanical strain on the cells of the bone-implant interface in an experimental system that allows growth of osteoblastic-like cells on the surface of an actual implant material.192
Additional limitations can be found in the methodologies mentioned previously. In many of the experiments, the imposed strains were not quantified. Some of the other studies that did report strain magnitudes used levels of strain that were either supraphysiological (>7000 µε)22 or subphysiological (<1500 µε)22 in nature.
The experimental techniques that have been discussed so far, such as strain gauges being applied to living bone, organ cultures, and cell culture, can provide illuminating data, but all of the techniques have drawbacks and sources of error. Strain gauges are technique sensitive and are difficult to use with biological tissues because of moisture, heat, irregularity of surface, and sometimes poor access to the application site. Their application to animal models can introduce further complications caused by the unpredictability of the behavior of the animal. Movements and loads artificially created when the animal is anesthetized may not give accurate data concerning physiological loads, but the animal may pull wires loose when it is awake.
Organ culture analysis may retain some of the spatial accuracy needed to test strain in the matrix; however, perfusion is necessary to maintain the organ tissue, and not much working time exists before the organ culture dies. Isolated cell culture models can give very useful information related to the release of certain biological mediators in response to the cells’ environment. However, again, organization has been lost, and this departure from the in vivo situation must be kept in mind when the results of such experiments are analyzed.
All of these experimental techniques are very valuable despite their individual drawbacks. When they are used in combination and their limitations are understood, helpful insight can be obtained and used to further understand functional loading and its biological ramifications.
Biomechanical Response
A compelling argument has been presented for strain-induced biological response of bone to mechanical load. The question remains: What controls the magnitude of strain imparted to the dental implant–bone interface?
Strain has been generically defined in relation to deformation and applied stress. The discussion of strain must be necessarily extended for biological structures. The mechanical properties of the trabecular and cortical bone found within the oral environment exhibit a high degree of variation as a function of load direction, rate, and duration. In addition, the structural density of the bone has a significant influence on its stiffness (modulus of elasticity) and ultimate strength. As such, the mechanical strain exhibited in bone is ultimately a function of the bone density.
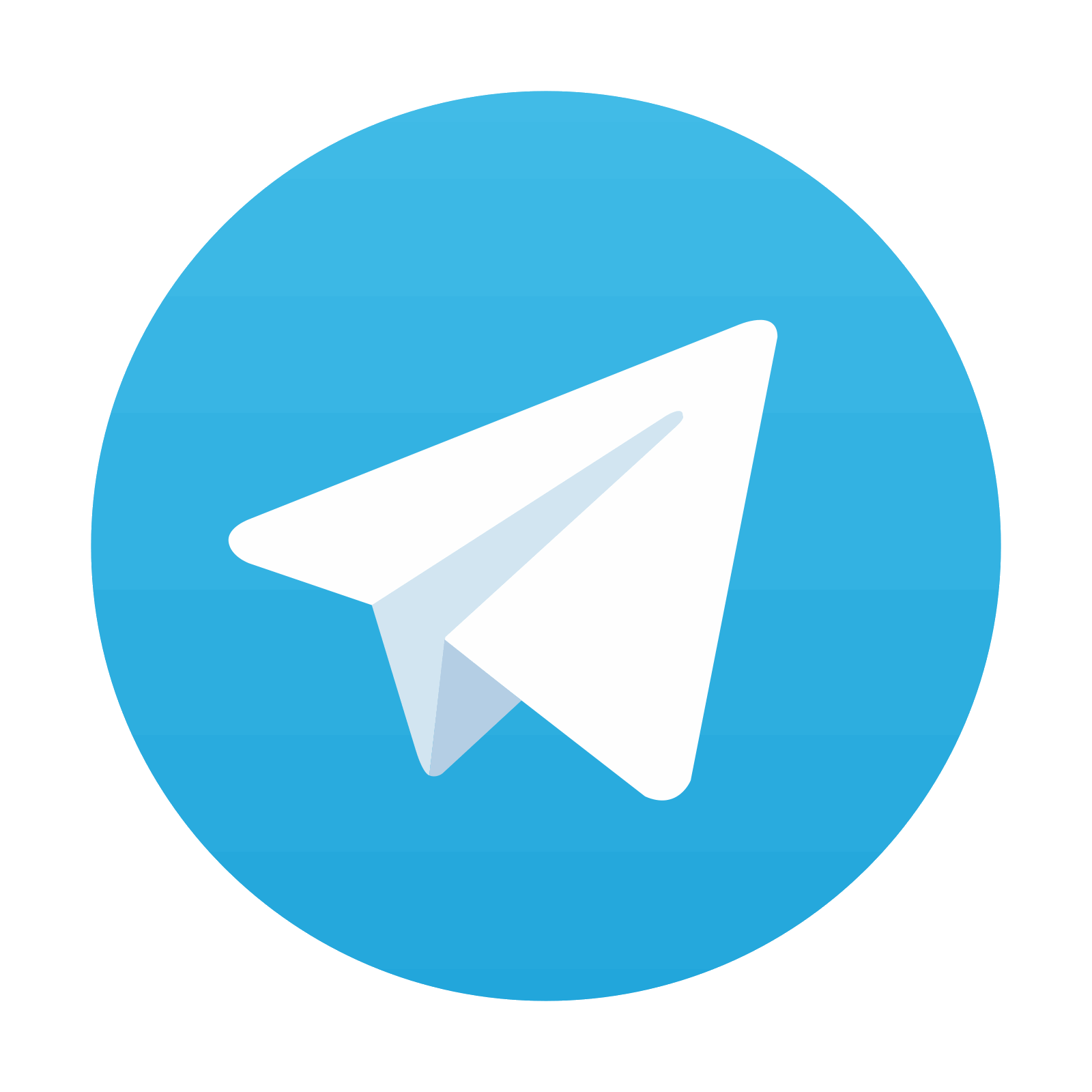
Stay updated, free dental videos. Join our Telegram channel

VIDEdental - Online dental courses
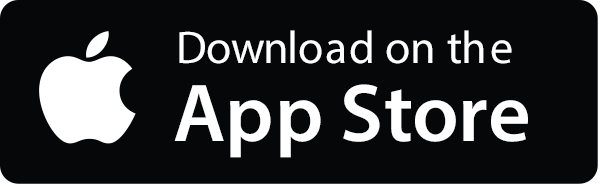
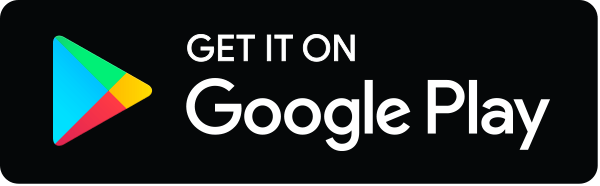