Abstract
Objectives
Additive manufacturing, which is more colloquially referred to as 3D printing, is quickly approaching mainstream adoption as a highly flexible processing technique that can be applied to plastic, metal, ceramic, concrete and other building materials. However, taking advantage of the tremendous versatility associated with in situ photopolymerization as well as the ability to select from a variety of preformed processible polymers, 3D printing predominantly targets the production of polymeric parts and models. The goal of this review is to connect the various additive manufacturing techniques with the monomeric and polymeric materials they use while highlighting emerging material-based developments.
Methods
Modern additive manufacturing technology was introduced approximately three decades ago but this review compiles recent peer-reviewed literature reports to demonstrate the evolution underway with respect to the various building techniques that differ significantly in approach as well as the new variations in polymer-based materials being employed.
Results
Recent growth of 3D printing has been dramatic and the ability of the various platform technologies to expand from rapid production prototypic models to the greater volume of readily customizable production of working parts is critical for continued high growth rates. This transition to working part production is highly dependent on adapting materials that deliver not only the requisite design accuracy but also the physical and mechanical properties necessary for the application.
Significance
With the weighty distinction of being called the next industrial revolution, 3D printing technologies is already altering many industrial and academic operations including changing models for future healthcare delivery in medicine and dentistry.
1
Introduction
The accessibility of 3D printers for both industrial and general public use has grown dramatically in the past decade. Global sales that include the devices, materials and services for industrial-scale to consumer-based printers have grown by an annual average of more than 33% over the last three years to a total of $4.1billion in 2014 . A significant driver of this growth is the fact that the early patents related to the additive manufacturing devices and processes have expired. This has opened the door for many start-up companies to develop new 3D printer devices that have pushed innovative design approaches while driving down the cost, in some cases well below $1000 for an entry-level printer. There are now more than 300 companies selling relatively inexpensive desktop devices, which currently is a category defined as units costing less than $5000 . This rapid evolution of the market has placed 3D printers not just in tremendously varied industrial settings but also in K-12 schools, public libraries, university classrooms and laboratories not to mention more and more commonly now, in homes. Some envision a future where virtual stores maintain only vast digital inventories that will allow customers to rapidly produce simple or complex products at a predictable, inexpensive price. Biomedical applications for 3D printing are one of the current growth leaders within a technology that overall is growing remarkably fast. We are already beginning to experience the integration of 3D printing in dental offices and laboratories. Although currently a fairly small component of dentistry, there are projections that the dental market for additive manufacturing processes is prime for explosive future growth. This paper provides an overview of the field of 3D printing involving polymeric materials with a focus on how developments in both device technology as well as the materials, whether in resin, filament or powder form, are combining to continue the impressive advance this rapidly moving technology.
The majority of 3D printing devices sold in 2014 for all medical uses were systems based on various material jetting and photopolymerization techniques [makepartsfast.com] with polymer-based printing accounting for the vast majority of the materials currently used in additive manufacturing market as a whole. These machines and the various polymeric materials that they accommodate or produce are suitable for creating a wide array of surgical guides and other tools as well as for the production of medical model implants, abutments, crowns, bridges and CT-imaged tissue replicas. New continuous rather than layered printing approaches allow structures of significant size to be printed in minutes and certain biocompatible-grade polymeric materials are approved for in-mouth placement. However, there remains a great amount of work to be done before 3D printed polymer-based materials are more widely implemented in dental practice. 3D printed ceramic and metallic constructs already are being used for implant, crown and bridge and direct other applications in dentistry. The opportunities for use of polymer-based 3D-printed materials across all aspects of dentistry are widespread but will depend on further improvements in the processing technologies as well as innovation in the materials being applied.
2
Additive manufacturing processes and materials
If taken loosely, the world of 3D printing encompasses a wide range of technologies. At its highest level, production of 3D parts can be sorted into three major categories – forming, subtractive or additive manufacturing. Forming involves the reshaping of a work piece without reducing or adding material with an example being vacuum forming molding. Subtractive manufacturing usually involves using cutting tools to remove unwanted material, as exemplified by the CNC milling of a precision part. As the name implies, additive manufacturing involves adding material rather than removing it to form the finished item. A clear advantage exists for additive processes when complex structures are required. The term 3D printing was originally associated with a specific additive processing technique but it is now commonly interchanged with the broader general designation of additive manufacturing. Rapid prototyping enabled by computer-aided design was conceived as a technique to allow engineers to efficiently and reliably convert virtual concepts to physical models and prototypic parts. The rapid production of physical models remains a key driver for the 3D printing market while the improvements in processing and the advancements in materials are now leading a transition toward 3D printing being used to produce readily customizable end-use parts that is expected to spur substantially greater growth. A significant component of the current market for printed structures involves the nimble creation of forms used to make molds that in turn are used to mass fabricate actual production parts. The additive manufacturing process is an effective means to produce limited-run, customized products with complex structure. In dentistry, the ability to satisfy the demand for patient-personalized models, tools and devices makes 3D printing a potentially very good fit with the profession ( Table 1 ).
SLA | Stereolithography apparatus |
DLP | Digital light projection |
CLIP | Continuous liquid interface production |
SLS | Selective laser sintering |
SHS | Selective heat sintering |
BAAM | Big area additive manufacturing |
FFF/FDM | Fused filament fabrication/fused deposition modeling |
LOM | Laminated object manufacturing |
While preformed polymeric materials in powder, filament and sheet form are used in 3D printing ( Fig. 1 ), several additive manufacturing also utilize the active polymerization of photo-sensitive resins. Since dentistry was an early adopter of photo-curing and remains heavily reliant on photopolymerization, it is natural that UV or visible light-based approaches to 3D printing will be utilized as dentistry takes advantage of this quickly developing technology. In fact, the photocuring as a methodology for 3D printing is particularly attractive for several reasons: high levels of build resolution, smooth part surfaces that do not typically require finishing processes, good z axis strength due to chemical bonding between layers, fast builds possible, and the ability to print clear objects. The initial layered material building approach was indeed photopolymerization based. The technology got its commercial start in 1986 when 3D Systems introduced the stereolithography apparatus (SLA) where specific surface regions of photosensitive liquid resin undergo localized polymerization by exposure to a rastered UV laser . A platform is first constructed to anchor the piece and support any overhanging structures. The x / y axis exposure of each distinct layer can be modified as the z axis incrementally evolves in the build process. Between each layer, the platform is lowered by 50 μm or less in higher resolution applications and 200 μm or more for parts with standard or lower resolution demands. When the part is complete, the excess resin is drained and can be reused. The formed parts are washed to remove excess resin and the support structures are physically removed. Depending on the resin material, a UV flood post-curing step may be included to raise conversion of the photopolymer. The finished parts generally have little surface roughness with appearances similar to that of molded parts. Final surface finishing can involve treatments with sealants, primers, paints or metallic coatings. A schematic representation of a stereolithography device is shown in Fig. 2 . 3D Systems also developed the requisite .STL or Standard Tessellation Language file format that permits three-dimensional orientation between slices that serves as the basis of all additive manufacturing processes although several other subsequent file formats have been developed .


In the SLA approach, the depth of cure, which ultimately determines the z axis resolution, is controlled by the photoinitiator and the irradiant exposure conditions (wavelength, power and exposure time/velocity) as well as any dyes, pigments or other added UV absorbers, since these processes typically involve UV sources in the form of UV lasers or UV LEDs. The use of UV absorbers allows the formation of transparent parts. The photo-induced layer thickness typically can range from approximately 50–200 μm. Step size is selected based on a balance between decreased build times and enhanced resolution. With micro-stereolithography and a UV-laser curing source, resolution of about 5 μm in the x / y plane and 10 μm in the z axis can be achieved . The use of a near-IR pulsed laser source in conjunction with a two-photon initiation strategy can create layers as fine as 100 nm, which is well below the light diffraction limit . Two-photon activation provides the ability to write lines or complex structures in three dimensions below a resin surface due to the focal patterning combined with the two-photon initiation mechanism . The two-photon approach typically requires alternative, large cross-section photoinitiators along with focused, high intensity irradiance at wavelengths approximately twice the absorbance maximum of the initiator to achieve reasonable efficiency; however, the approach remains practically limited to micro-machining scale applications in terms of part production, such as lab-on-chip devices, microfluidic devices, photonic crystals, optical waveguides, nerve scaffolds and many others. Surface-patterned exposure from digital light projection (DLP) sources and using high power LED sources essentially allows any selected portion of the entire x / y workspace to be exposed simultaneously as opposed to dynamic writing with a condensed laser beam. Even though high laser scanning velocities are employed in the SLA approach, the ability to simultaneously photocure all portions of a given slice with DLP significantly speeds cycle times between layers. The SLA or DLP techniques can be used with a wide variety of monomers and resin systems. An example of the versatility in SLA monomer selection is demonstrated with the complex UV-curable hybrid resin prepared with both radical activated acrylates and cationic initiated epoxy monomers as a route to fast photocuring multi-phase polymers with low polymerization shrinkage . The polymerization-induced shrinkage as the photopolymer is formed when coupled with the thermal expansion/contraction leads to stress development that can produce warped parts. Strategies based on both the material selection and the exposure protocol can be developed to largely mitigate the development of residual stresses and heated resin baths are also possible to reduce resin viscosity while increasing polymerization speed and degree of conversion in the photopolymer.
Ideally, the monomers (typically comonomers and reactive oligomers) used in photopolymerization-based part printing should be of relatively low to modest overall viscosity (viscosities either too low or too high introduce problems), capable of rapid polymerization and yield crosslinked polymers with properties suited to the demands imposed by the target application. Just as in the UV-curable coatings industry, this means that acrylates and epoxy monomers are most commonly encountered as photo-based printing materials although vinyl ether-functionalized monomers and other monomer types are used as well. Also related to the UV coatings industry, relatively high photoinitiator concentrations (often up to 3–5 wt%) are used to achieve fast polymerization and limited depth of cure. With the fast curing monomers and short irradiation exposures, significant amounts of residual initiator remain and this permits parts to be post-cured in a UV oven to promote completion of the curing process. Some work with relatively viscous resin systems has demonstrated that the sacrificial support structure for overhanging elements or freely moving aspects of the part design can be eliminated but this also increases the cycle times between layers. An alternate materials-based approach to creating support structures utilizes a thermally reversible Diels–Alder network swollen with a photo-crosslinkable acrylate monomer. This strategy allows a permanent photo-patterned structure to be developed and then released from the supporting matrix by raising the temperature to cause reverse gelation in the unexposed regions .
The mechanical strength of the parts produced by the SLA approach is somewhat limited by the viscosity restrictions generally imposed on the resins as well as the preference for faster curing acrylate monomers rather than analogous methacrylates, which offer higher strength polymers and better biocompatibility compared with acrylates. However, since the photopolymerization of each new layer is intimately connected with the prior layer, this leads to good strength and minimal anisotropy in the structure and properties of SLA printed parts. Initiators should be matched well to the irradiation source and have high molar absorptivity to achieve high photocuring efficiency as well as a shallow depth of cure.
A recent development in UV-based 3D printing involves continuous liquid interface production (CLIP) that utilizes a bottom-up building approach that is facilitated through a well-controlled oxygen inhibited dead-zone that avoids attachment of the 3D part to an oxygen-permeable curing window . The window ( Fig. 3 ) is a thin, amorphous Teflon film with high chemical resistance and gas transport potential, very low refractive index and excellent optical clarity that extends into the UV range. As with SLA, the choice of photo-active resins is fairly broad; however, the viscosity and reactivity of the monomers are critical here since even small variations affect the physical and kinetic aspects of oxygen diffusion within the resin that dictate the dimension of the dead-zone, which is approximately 20–30 μm as a practical minimum. Within this region, the diffused oxygen completely consumes the initiating radicals to avoid adhesion of the part. The rate of resin replenishment in this dead-zone, the initiation efficiency and the resin reactivity all combine to determine the rate at which the part can be formed in continuous rather than layer-by-layer fashion. The platform elevation rate can vary according to the area of exposure at any stage of the build process. The resulting part resolution is inversely related to the print speed but parts with feature size down to 10’s of μm can be formed with z axis print speeds of 25–1000 mm/min, which considerably outpaces the production efficiencies associated with step-wise layered building techniques that typically take several hours with conventional SLA techniques.

MultiJet (or PolyJet) printing utilizes another UV-cured printing format that can produce smooth parts in highly complex geometries that do not require surface finishing since layer dimensions below 20 μm are possible. The UV-curable polymeric materials are applied only where desired for the design and since multiple print heads can be used, a wax or other gel-like supporting material can be co-deposited for subsequent removal by heating or water washout . Since multiple printing heads are simultaneously available ( Fig. 4 ), free variations in blended color or even different building materials with different properties can be spatially designated including the formation or structures with spatially graded properties . For jetted inks, a shear-thinning, non-Newtonian fluid is a desirable component that provides some restriction in the material choice. Ink viscosity can vary and be compensated for with either reactive diluent additives or heated print heads. Rather than using wax as a support material, objects can be formed entirely from jetted wax and then used directly for investment casting applications, which is often encountered in dentistry and in jewelry design applications. Much work has already developed a range of UV-curable inkjet inks for 2D printing applications. These formulations are typically composed of monomers, oligomers, colorants (pigments or dyes dispersed or dissolved in the reactive carrier), photoinitiator(s) and other additives. A UV-curable ink applied as an oil-in-water emulsion has been used to create intentional well-controlled porosity in parts upon curing followed by evaporation of the aqueous phase. With a co-continuous phase structure in the ink, interconnected pores can be obtained in printed parts that permit infiltration to achieve, for example, conductive materials . Inks can contain nano-fillers such as silica, clay of suitable dimension to be accommodated within a jetted ink droplet to provide both viscosity control during deposition as well as reinforced mechanical properties in the final part . A leveling blade can be used between layers to assure uniform build layers prior to exposure to the UV curing lamp and z axis stage translation in preparation for the next layer. The parts produced are dimensionally accurate and can be robust in their mechanical properties; however, the build orientation can significantly affect elastic modulus and fracture stress but tensile strength is relatively insensitive to print direction . However, as with all 3D printing approaches, the degree of isotropic mechanical property character can vary greatly based on the material used with any given building process.

Selective laser sintering (SLS) was introduced soon after the SLA technique but it employs primarily semi-crystalline, particulate thermoplastic prepolymer as the building material. The technique relies on two energy sources accomplish part production. First a bed of polymeric particles is preheated close to the melting transition and above the temperature necessary for recrystallization during the cooling cycle. The preheated powder bed limits the energy input necessary from the laser to cause sintering, which avoids large thermal differentials that would otherwise result in part distortion. Localized thermal sintering of the particles is achieved by the controlled additional energy input by a high-power CO 2 laser, which traces the 2D layer design that fuses exposed particles together within the layer as well as connects it to the previously scanned underlying layer ( Fig. 5 ). The laser power (up to 20–50 W), beam size (typically about 0.5 mm), beam speed and spacing between scans (both physical, known as hatch spacing, and temporal) needs to be carefully controlled to balance effective sintering while avoiding polymer property degradation that comes with overheating . The sintering steps are interspersed with application of an incremental layer of prepolymer powder of approximately 100 μm applied by a roller or blade. Since the polymer powders are approximately 30–90 μm in dimension, this means only about 2–4 particles represent a layer thickness. This minimizes the extent of indirect heating based on thermal conductivity to provide effective sintering. The particles must be free flowing since layers receive no further compaction. Therefore, particle size, shape and free packing density are critical factors in the material design along with the thermal behavior. Spherical particles flow more easily and pack more densely than irregular shapes so ground polymer processing is problematic since it creates irregular particle shape and a broad distribution of particle size. Particles that are too small create processing difficulties due to either excessive cohesion or electrostatic repulsive forces. After the part is completed, the entire building chamber is cooled slowly to maximize polymer crystallization to provide added strength as well as to reduce stress development and improve dimensional accuracy, which can be improved further by incorporation of shrinkage modeling into the initial part design . The unsintered powder serves as the physical support for the part throughout the process. The free powder is removed at the end of the build cycle and it can generally be reused albeit with concerns of material alteration related to oxidation (which can be mainly avoided by an inert gas purging), thermal degradation and even increases in polymer molecular weight (with changes in many properties including reduced polymer crystallinity) during the extended heating process. This means that recycled polymer typically is mixed with virgin powder for subsequent use . Rather than the aging effects associated passive heating, a separate study investigated PA12 material alterations resulting from the higher temperatures as a result of direct laser exposure at varied power levels. The results were that higher laser power resulted in PA12 chain scission as evidenced by lower viscosity and lower values for elongation at break .

The scanned laser produces a melt zone within the polymer powder bed that has a comparable radius in both the horizontal and vertical dimensions. Within this zone, depending on particle size and laser power, adjacent particles may be completely melted or just liquefied at the particle surface with gravity and capillary forces serving to produce consolidation . In addition to the more dense core within the scanned beam path, the sintered zone extends to a more loosely bound and less dense boundary layer that was exposed to less power and lower temperatures based on thermal conductivity . When the processing conditions are well matched to the polymeric material , including the hatch spacing between adjacent scans in the x / y plane, reasonable mechanical strength and good toughness can be achieved with certain materials. One approach to reaching increased part density, minimizing defects and improving mechanical properties involves the application of pressure in the build chamber . Polyamide (PA12 or nylon 12) represents the vast majority of the current commercial SLS materials market. Alternative materials include other polyamides (PA6, PA10, PA11), poly(acrylonitrile/butadiene/styrene) (ABS), polystyrene (PS), polycarbonate (PC) and work continues to develop polypropylene, high-density polyethylene, polyether ether ketone (PEEK) among others for use in polymer powder form. Significantly higher powder bed heating temperatures are necessary to allow use of high performance polymers such as PEEK. These high powder processing temperatures (∼350 °C) limit potential recycling of the non-fused PEEK powder, which greatly increases the production expense, while also excluding its use with most commercial SLS devices .
Semi-crystalline polymers are primarily used with the intrinsic selection criteria ideally including a broad process temperature window between polymer melting upon heating and recrystallization upon cooling, a narrow melt transition, and a high melting enthalpy to minimize unwanted sintering associated with thermal conductivity . The surface tension of the particle must also be sufficient to avoid interparticle fusion even though the powder is maintained far above its glass transition temperature for extended intervals. The polymers can be selected to yield parts that are glassy or elastomeric. The preheating and laser sintering conditions can be empirically modified to account for differences in melting temperatures of materials as well as also being able to work with amorphous polymers that display elevated glass transition temperatures. Amorphous polymers such as polycarbonate or polystyrene tend to yield weaker, more porous structures than the semi-crystalline polymer powders , although relatively brittle polystyrene parts can be strengthened and toughened by substitution of poly(styrene-acrylonitrile) or ABS . However, amorphous polymers do not undergo the significant dimensional contraction associated with polymer crystallization as the process temperature is reduced . In some situations, a more porous structure may be desired so that a secondary infiltration with an epoxy resin or wax can be used to provide parts with much enhanced mechanical properties or varied surface finish . While poly(methyl methacrylate) is used widely in dentistry and many other amorphous commodity polymer applications, PMMA has only recently been adapted for use in 3D printing . As with all the polymer powders, very rigorously controlled, high-grade material stocks are required. So while the palate of polymers for use in SLS is growing, it is orders of magnitude smaller than the choices of polymers available for injection and extrusion molding applications. A range of fillers, such as silica, aluminum, carbon fiber and glass can also be incorporated into the powdered polymer to further modify the appearance and properties of the printed parts. One study has demonstrated that the SLS processing introduces an orientation bias of micro-scale carbon fiber filler in PA parts . Silica can be added separately to polymer powders to improve flow properties. Achieving uniform distribution of filler in a mixed powder system where differences in particle size and density are involved makes this nontrivial. The factors that govern a successful material are quite complex in terms of both the polymer powder production and its interaction with the SLS process . The current state-of-the-art in SLS is that a given material will have significantly different mechanical properties based on how it is oriented , and processed within a given device and that because of the sensitivity of the materials to the process conditions, the same material used on different devices can produce quite varied results . Certain materials, PA12 being a good example, are more forgiving in that regard but the transition from printed structures used mainly as models toward 3D printed functional parts remains a challenge; however it is a target toward which definite progress is being made. A technology known as Selective Heat Sintering (SHS) utilizes a thermal printhead rather than a laser to fuse the surface of a powdered thermoplastic into patterned, layered structures analogous to the SLS approach.
Another AM technique that relates to both SLS and MultiJet printing is the binder jetting process. This method originally gave rise to the term “3D printing” that subsequently has been broadened to include all the additive manufacturing approaches described here. A liquid binder is delivered with high spatial resolution to a powder bed surface from a print head. The binder connects just the exposed particles together either through solvent welding or chemical reaction with no thermal processing necessary, which improves building efficiency since no cooling cycle is involved and the unexposed powder stock can be freely reused. The powder can be polymer or many other materials and a range of binders can be employed based on the powder used. With some materials, a dilute polymer solution is used as the binder. The layers need to remain small to allow effective binding between incremental layers while also achieving reasonable x / y resolution. The choice of polymeric powder materials can be much broader than that associated with SLS since the constraints involved with thermal processing windows are largely avoided; however, for solvent welded parts or in applications with solvent-carried adhesives, there are obvious solvent resistance considerations. The ability to use multi-print heads dispensing binder of different colors provides a means to produce blended multicolored articles; however, the final parts have substantial porosity, which may necessitate infusion of a reactive resin or wax to provide suitable surface finish and strength .
Fused filament fabrication (FFF) (or fused deposition modeling – FDM) was developed in the early 1990s as another 3D printing approach that like SLS uses preformed polymer as the building material. However, in this case, the processing energy input is involved at the pre-deposition stage to obtain a polymer melt material that can be applied through a fine print head or nozzle ( Fig. 6 ). As such, this method is analogous to conventional extrusion or injection molding except that molds are unnecessary. Heated build chambers can be used to minimize the thermal distortion associated with non-uniform cooling. The FFF technique produces somewhat greater anisotropy in terms of properties compared with the SLA and SLS printing processes. One approach to address this directional disparity in strength and toughness that results from interlayer bonding being less than intra-layer strength relies on a post-print processing with exposure to gamma radiation to induce crosslink formation within and between FFF printed layers . Since polymer melt extrusion in the FFF method requires processible prepolymers, the ionizing radiation provides a route to convert a thermoplastic polymer to a thermoset final printed part that along with more homogeneous mechanical properties also displays greater solvent resistance that may be important in biomedical and other applications. A materials-based approach that circumvents the processing barrier associated with thermoset polymeric materials in the FFF technique relies on the thermally reversible Diels–Alder reaction to allow heated flow of the polymer followed by covalent bond formation upon cooling as a means to provide reinforcement between applied layers in 3D builds .
Multiple print heads can be accommodated with FFF devices to permit co-printing of temporary support material for complex overhanging structure as well as use of multicolor or different build materials to be used within a single part; however, unlike the MultiJet process, intimately blended colors or materials cannot be accommodated in the design. Most of the commercial FFF devices are used with ABS or PLA thermoplastic materials delivered as fibers from spools. Other material options include polycarbonate, polyamide, high-impact polystyrene, polyetherimide, polyoxymethylene, polyphenylsulfone and others. Recent work has further expanded the materials palette to include other polymer blends as well as ultra-high molecular weight polyethylene . Due to the greater materials tolerance of the FFF approach as compared with SLS processes, even recycled commodity polymer stocks can be considered for use . As with the jetted printing inks, nano-fillers can be included to induce the shear thinning character but the continuous filament approach uniquely allows high aspect ratio microscopic fillers to be used to provide greatly enhanced polymeric mechanical strength based on alignment within the filament. Silicon carbide and carbon fiber with dimensions of 0.65 μm × 12 μm and 10 μm × 220 μm, respectively, have been used with an epoxy-based resin, which relies on a post-print thermal treatment to achieve final cure, to produce printed parts with strength and modulus results that significantly surpass the mechanical properties of conventionally molded samples of the unfilled base resin . The thermal treatment when combined with well-design printing layout is probably also responsible for the printed parts showing little anisotropic character. Modeling considerations of factors such as the pressure drop and bead cooling as the polymer melt leaves the nozzle and condenses with adjacent previously deposited material has received considerable attention . Despite these technical details, the relatively straightforward FFF ‘melt-apply-solidify’ approach has lead to this being by far the most common version of 3D printing and it represents the vast majority of consumer-based low cost devices. An alternate approach related in principle to FFF uses a resin with sufficient viscosity based on the resin itself or due to added filler that yield an extrudable filament that can be rapidly solidified in place based on gelation reaction, drying or photopolymerization. An example of this is the free-form building of continuous microstructures based on resin extrusion coupled with UV curing .
As an example of what the future of fused deposition 3D printing may include, the US Department of Energy, which is interested in efficiency and energy aspects related to manufacturing, printed a full-size, working replica of a Shelby Cobra as an electric car that was displayed at the 2015 North American International Auto Show in Detroit . The frame, body and other parts (500 lbs in total) were printed from ABS thermoplastic containing 20% carbon fiber using the big area additive manufacturing (BAAM) facility at Oak Ridge National Laboratory where the build space is 20 × 8 × 6 ft and the material deposition cross section is well above the conventional filament scale. A calculation of the energy used to print the car was significantly less than that associated with typical auto manufacturing practices.
A recent marriage between filament deposition 3D printing and electrospinning has further expanded the possibilities for the preparation of reinforced tissue engineering scaffold structures among other applications. By moving the collector plate in the same fashion as FFF techniques, the random coiling nature of the electrospinning process can be circumvented to form very well defined continuous patterned structures . Laminated object manufacturing (LOM) is yet another layered manufacturing technique that uses a variety of materials including polymer-based foils in layer-by-layer application process interspersed with cutting with a blade or laser to achieve spatial patterning within each of the laminated film layers . Thus, this approach represents a compromise between additive and subtractive manufacturing strategies in the building of three dimensional structures.
The areas of 3D printing in bioengineering including bioprinting, which involves printing structures that include cells, DNA and other bioactive components as integral parts of the building process, are growing at a remarkable rate . Labs everywhere are printing prototypic organs including heart valves, ears, artificial bone, joints, menisci, vascular tubes and skin grafts. The structural and compositional complexity associated with many types of tissue mimics greatly complicates both the programming and the printing . Even the tremendous challenge of achieving viable vascularized tissue constructs is potentially enabled by the additive processing approach . In tissue engineering, the ability to reproducibly print the physical aspects of a tissue scaffold or other constructs for use in complex cell-based studies means that this physical parameter can be removed as a variable while the chemical, mechanical or other properties of a scaffold are systematically probed. Alternatively, physical features such as pore structure in a given scaffold material can be manipulated at will . Poly(latic acid) is commercially available for SLS and FFF printing applications and because of the widespread use of PLA in other forms in human, animal and cell-based studies, it is a popular choice for biomedical related polymer printing . Polycaprolactone is also being evaluated as a biodegradable printed scaffolding material . Due to the specific material requirements associated with scaffolds for bioengineering, microfluidic devices, drug delivery vehicles and many other biological applications, the medical field has actually significantly expanded the number of new polymers that have been examined for use in 3D printing applications .
Additive manufacturing of polymeric biomedical materials are also being applied to dentistry with patient-specific constructs used in clinical applications beginning to emerge . This type of hard and soft tissue repair will certainly expand and there are predictions of printed replacement teeth but as with the other organ printing considerations, implementation of these prospects remains an aspiration on the horizon. Current mainstream polymer-based 3D printing applied to clinical dental practice include dental wax-ups, orthodontic patterns, crown and bridge molds, which can offer quicker turnarounds along with local control of the model processing. Presently, 3D printing is being used in dental laboratories around the world. Restorative labs are using it to make patterns for fixed prosthodontics, surgical guides, and complete removable dentures. Based on individual patient data, implant drill guides as well as thermo-formable orthodontic patterns and aligners are also in use in dental offices with additive manufacturing devices designed exclusively for dental and medical applications being very actively promoted to the dental profession. Models of mandibles and other complex tissue structure can be realized relatively rapidly based on the 3D data obtained by digital scanning techniques including laser scanning, computerized tomography (CT) and magnetic resonance imaging (MRI) . Materials have been specifically designed to produce life-like colors and textures for teeth and gingiva in models. Recent advances in 3D printing speed while retaining the potential for high spatial accuracy and resolution will only further expand these opportunities. One such opportunity is demonstrated by the option to make printed structures that include bioactive components. As an example, a drug (nitrofurantoin) as well as hydroxy apatite were blended into PLA to obtain an extruded filament with up to a 30% mass fraction of drug that could be processed by FFF to create a device that prevented surface-associated and planktonic growth of Staphylococcus aureus . The development of 3D printed drug delivery devices with spatial variations in drug loading and material properties is an area of growing interest that will likely find application in dentistry . The accuracy of 3D printed dental models relative to plaster casts has been documented . However, a recent study focused on the dimensional fidelity of printed die spacers relative to the input computer-aided design parameters for crown fabrication demonstrated some inaccuracies and inconsistencies in print-producing these sub-100 μm details . As the materials, the 3D build design software and the hardware associated with the building processes continue to improve, obviously more mainstream as well as specialized applications of 3D polymer-based printing in dentistry will continue to develop with further expansion into both dental labs and dental practices.
It should also be noted that there are well-designed studies to compare the dimensional accuracy, mechanical properties, surface roughness, build speed and materials cost across multiple 3D printing platforms . This demonstrates that there are advantages and disadvantages associated with each approach. As the penetration of additive manufacturing continues to grow and evolve in the industrial world as well as in the varied arenas of dental/biomedical materials and tissue engineering, quality standards associated with the wide array of 3D printing processes and materials becomes of greater concern. To address this issue, ASTM International Committee F42 on additive manufacturing technologies has been engaged and there is an ASTM standard (F2792-12a) on the standard terminology for additive manufacturing technologies . The National Institute for Standards and Technology has proposed a standard AM test artifact . This artifact would permit independent, objective assessment of build quality based on standardized measurements. The proposed artifact would be large enough to provide features near edges of build platforms as well as central locations and contain practical features such as flat surfaces, ramps, arcs, thin walls, holes and protruding features over a broad range of length scales.
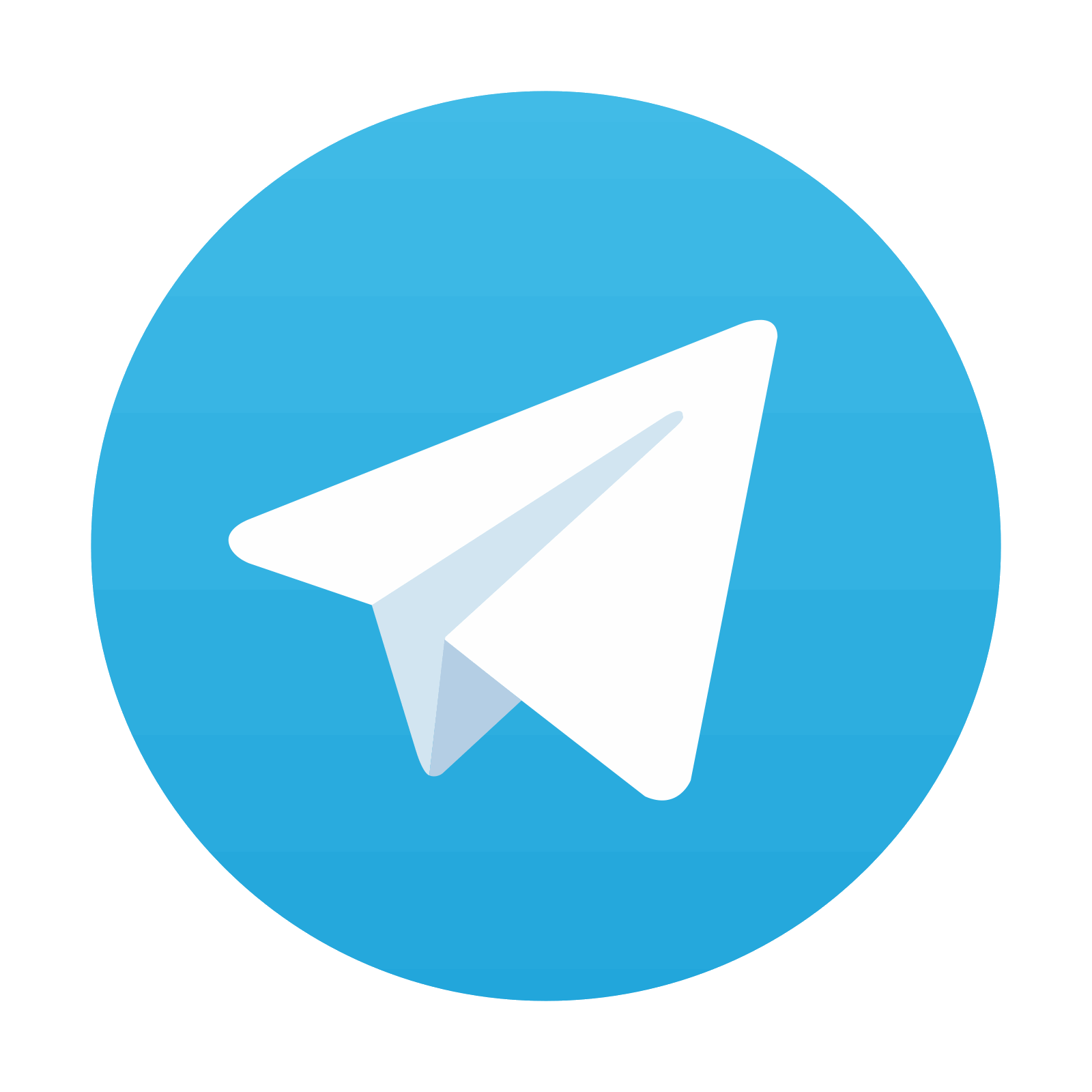
Stay updated, free dental videos. Join our Telegram channel

VIDEdental - Online dental courses
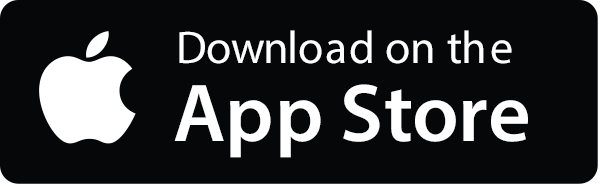
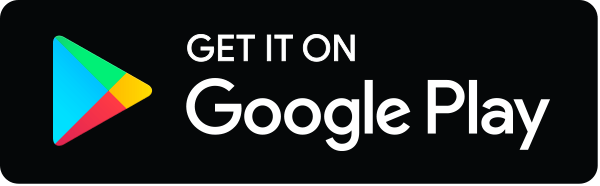