Chapter 26 Bone Physiology, Metabolism, and Biomechanics
Consistent success with implant-supported prostheses requires a thorough knowledge of the physiology, metabolism and biomechanics of bone as a tissue, and bones as musculoskeletal organs. Bone is a vital mineralized tissue and bones are unique morphologic organs, composed of calcified and soft tissues that provide structural and metabolic support for a wide variety of interactive functions (Figure 26-1).
Understanding the clinical manipulation of bone begins with an appreciation of the fundamental genetic and environmental mechanisms of osseous development and adaptation. The genome codes for growth factors, ischemic agents, vascular induction/invasion mechanisms, and mechanically induced inflammation. These biological mechanisms interact with the physical factors of diffusion limitation and mechanical loading to produce bone morphology (Figure 26-2). Fundamental principles control the quality and quantity of bone that directly and indirectly supports stomatognathic function. A firm grasp of the modern concepts of bone physiology, metabolism, and biomechanics is an essential prerequisite for innovative clinical practice. These principles are an objective basis for designing a realistic treatment plan that has a high probability of meeting the esthetic and functional expectations of the patient.
Bone is a dynamic structure that is adapting constantly to its environment. Because the skeleton is the principal reservoir of calcium, bone remodeling (physiologic turnover) performs a critical life support role in mineral metabolism (Figure 26-3). Collectively, bones are essential elements for locomotion, antigravity support, and life-sustaining functions such as mastication. Mechanical adaptation of bone is the physiologic basis of stomatognathic reconstruction with implant-supported prostheses. A detailed knowledge of the dynamic nature of bone physiology and biomechanics is essential to enlightened clinical practice.
OSTEOLOGY
In defining the physiologic basis of orthodontics, the initial consideration is bone morphology (osteology) of the craniofacial complex. Via the systematic study of a personal collection of more than 1000 human skulls, Spencer Atkinson1 provided the modern basis of craniofacial osseous morphology as it relates to the biomechanics of stomatognathic function. A frontal section of an adult skull shows the bilateral symmetry of bone morphology and functional loading (Figures 26-4 and 26-5). Because the human genome contains genes to pattern the structure of only half of the body, the contralateral side is a mirror image. Consequently, normal development of the head is symmetrical. Thus unilateral structures are on the midline, and bilateral structures are equidistant from it. As shown in Figure 26-5, the vertical components of the cranium tend to be loaded in compression (negative stress), and the horizontal components are loaded in tension (positive stress). From an engineering perspective, the internal skeletal structure of the midface is similar to that of a ladder: vertical rails loaded in compression connected by rungs loaded in tension. This is one of the most efficient structures for achieving maximal compressive strength with minimal mass in a composite material.
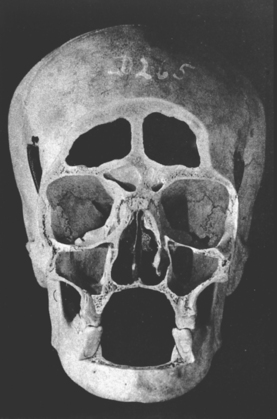
Figure 26-4 Frontal section of a human skull in the plane of the first molars.
(From Atkinson SR: Balance: the magic word, Am J Orthod 50:189, 1964.)
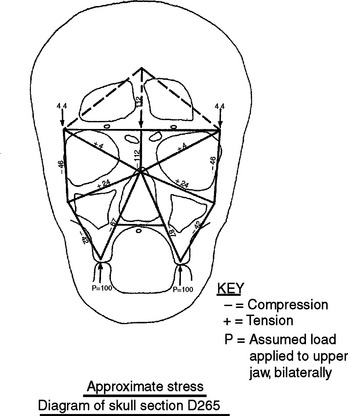
Figure 26-5 Two-dimensional vector analysis of stress in the frontal section of the human skull depicted in Figure 26-4. Relative to a bilateral biting force of 100 arbitrary units, the load is distributed to the vertical components of the midface as compressive (negative) stress. The horizontal structural components are loaded in tension. In a nongrowing individual the stress across the midpalatal suture is 0. When masticating, loads increase and the midpalatal suture is subjected to a tensile load, resulting in an increase in maxillary width.
(From Atkinson SR: Balance: the magic word, Am J Orthod 50:189, 1964.)
Differential Osteology of the Maxilla and Mandible
Although equal and opposite functional loads are delivered to the maxilla and mandible, the maxilla transfers stress to the entire cranium, whereas the mandible must absorb the entire load. Consequently, the mandible is much stronger and stiffer than the maxilla. A midsagittal section through the incisors (Figure 26-6) and a frontal section through the molar region (Figure 26-7) show the distinct differences in the osseous morphology of the maxilla and mandible. The maxilla has relatively thin cortices that are interconnected by a network of trabeculae (see Figures 26-4, 26-6, and 26-7). Because it is loaded primarily in compression, the maxilla is structurally similar to the body of a vertebra.
The mandible, however, has thick cortices and more radially oriented trabeculae (see Figures 26-6 and 26-7). The structural array is similar to the shaft of a long bone and indicates that the mandible is loaded predominantly in bending and torsion. This biomechanical impression based on osteology is confirmed by in vivo strain gauge studies in monkeys. Hylander2,3 demonstrated substantial bending and torsion in the body of the mandible associated with normal masticatory function (Figure 26-8). A clinical correlation consistent with this pattern of surface strain is the tendency of some humans to form tori in the areas of maximal bending and torsion (Figure 26-9). The largest tori are on the side on which the individual habitually chews (preferential working side).
Temporomandibular Articulation
The temporomandibular joint (TMJ) is the principal adaptive center for determining the intermaxillary relationship in all three planes of space. Figure 26-10 shows optimal skeletal development consistent with normal morphology of the TMJ. Figure 26-11 shows aberrant skeletal and dental relationships consistent with degeneration of the fossa and mandibular condyle (i.e., the enlarged mushroom shape of the condylar process, the roughened topography of the articulating surfaces, the loss of articular cartilage and subchondral plate). Progressive degeneration or hyperplasia of one or both mandibular condyles may result in substantial intermaxillary discrepancies in the sagittal, vertical, and frontal dimensions. Adaptation of the TMJ allows for substantial growth change to occur without disturbing the intermaxillary relationship of the dentition (e.g., Class I occlusion remains Class I). In the adult years the intermaxillary relationship continues to change but at a slower rate. The face lengthens and may rotate anteriorly as much as 10 mm over the adult lifetime.4 The mandible adapts to this change by lengthening and maintaining the intermaxillary dental relationship (Figure 26-12). However, if the TMJs of an adult undergo bilateral degenerative change, whether symptomatic or not, the mandible can decrease in length, resulting in a shorter, more convex face (Figure 26-13).
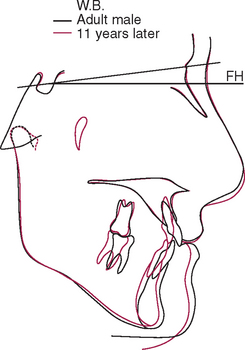
Figure 26-13 Superimposed cephalometric tracings of a middle-aged man over an 11-year period. Although the soft tissue changes are similar to those seen in other adult males (see Figure 26-12, A), the less prominent mandible and more convex skeletal profile are consistent with a shortened mandible. This man had a history of bilateral temporomandibular internal derangement that progressed to crepitus. However, despite the substantial change in facial form and occlusal compensation, no appreciable pain was reported.
Within physiologic limits, the TMJ has remarkable regenerative and adaptive capabilities allowing for spontaneous recovery from degenerative episodes (Figure 26-14). Unlike other joints in the body, the TMJ has the ability to adapt to altered jaw structure and function. After a subcondylar fracture, the condylar head is pulled medially by the superior pterygoid muscle and resorbs. If the interocclusal relationship is maintained, a new condyle forms from the medial aspect of the ramus and assumes normal function. Unilateral subcondylar fractures usually result in regeneration of a new functional condyle with no significant deviation of the mandible.5 However, about one fourth of subcondylar fractures result in a mandibular deviation toward the injured side, resulting in an asymmetrical Class II malocclusion with a midline deviation. Another sequela of mandibular trauma is internal derangement such as a unilateral closed lock (a condyle distally displaced relative to the disk). If the range of motion is reduced in a growing patient, the compromised function may inhibit mandibular growth, resulting in a cant of the occlusal plane. Progressive dysfunction and pain may ensue, particularly when associated with occlusal trauma. Reestablishing normal bilateral function allows the compromised condyle or condyles to adapt favorably.
BONE PHYSIOLOGY
Specific Assessment Methodology
Mineralized Sections
Fully mineralized specimens are superior to routine demineralized histologic sections for most critical analyses of teeth, periodontium, and supporting bone because fully mineralized specimens experience less processing distortion. Furthermore, the inorganic mineral and organic matrix can be studied simultaneously.6–8,22 For tissue-level studies, sections 100 μm thick are appropriate because they can be studied by means of several analytic methods. Even without bone labels, microradiographic images of polished mineralized sections provide substantial information about the strength, maturation, and turnover rate of cortical bone (Figure 26-15, A). Reducing the thickness of the section to less than 25 μm considerably enhances cellular detail and resolution of bone labels. Specific stains are useful for enhancing the contrast of cellular and extracellular structures. The disadvantages of thin mineralized sections are (1) bone labels quench more rapidly and (2) tissue density is inadequate for microradiographic analysis.
Polarized Light
Birefringence of polarized light (see Figure 26-15, B) has particular biomechanical significance. The lamellar fringe patterns revealed with polarized light indicate the preferential collagen orientation within the matrix.23 Most lamellar bone has alternating layers of collagen fibers at right angles. However, two specialized collagen configurations can be seen in the same or adjacent osteons: (1) longitudinally aligned collagen fibers efficiently resist tension and (2) transverse or circumferential collagen fibers are preferential supports for compression.24 Loading conditions at the time of bone formation appear to dictate the orientation of the collagen fibers to best resist the loads to which the bone is exposed. The important point is that bone formation can adapt to different loading conditions by changing the internal lamellar organization of mineralized tissue.
Fluorescent Labels
Administered in vivo, calcium-binding labels are anabolic time markers of bone formation. Histomorphometric analysis of label incidence and inter-label distance is an effective method of determining the mechanisms of bone growth and functional adaptation (see Figure 26-15, C). Because they fluoresce at different wavelengths (colors), six bone labels can be used: (1) tetracycline (10 mg/kg, bright yellow); (2) calcein green (5 mg/kg, bright green); (3) xylenol orange (60 mg/kg, orange); (4) alizarin complexone (20 mg/kg, red); (5) demeclocycline (10 mg/kg, gold); and (6) oxytetracycline (10 mg/kg, dull or greenish yellow). The multiple-fluorochrome method (sequential use of a variety of different colored labels) is a powerful method of assessing bone growth, healing, functional adaptation, and response to applied loads.7,25
Microradiography
High-resolution images require polished sections approximately 100 μm thick. Differential radiographic attenuation shows that new bone is less mineralized than mature bone. Newly formed bone matrix is osteoid and requires about 1 week of maturation to become mineralized bone matrix. Depending on the collagen configuration of the bone matrix, osteoblasts deposit 70% to 85% of the eventual mineral complement by a process called primary mineralization.7,24 Secondary mineralization (mineral maturation) completes the maturation process in about 8 months by a crystal growth process (see Figure 26-15, D). Because the strength of bone tissue is related directly to mineral content, the stiffness and strength of an entire bone depends on the distribution and relative degree of mineralization of its osseous tissue.26 The initial strength of new bone is the result of the cell-mediated process of primary mineralization, but its ultimate strength is dictated by secondary mineralization, which is the physiochemical process of crystal growth. This concept has important clinical value in orthodontics. Fully mineralized lamellar bone (i.e., bone in steady state with respect to modeling and remodeling) is expected to be less susceptible to relapse tendencies than its woven and composite bone predecessors.6,27 After active orthodontic therapy, retaining dental corrections for at least 6 to 8 months is important to allow for mineral maturation of the newly formed bone (Figure 26-16).
Endosseous implants can be placed in the nasal bones of rabbits to serve as anchorage units to load the nasal suture. Slow sutural expansion (Figure 26-17) produces high-quality lamellar bone along the osseous margins of the suture and the periosteal (superior) surface of the nasal bones (Figure 26-18). Compression of the nasal bones (see Figure 26-17, A) is manifested by resorption along the margins of the suture (Figure 26-19). Compressive and tensile loading of the suture are associated with extensive bone modeling and remodeling of the adjacent bones. Sutural adaptation to physiologic and therapeutic loads is associated with a regional acceleration of modeling and remodeling in the adjacent bones.
The PDL is the adaptive connective tissue interface between a tooth and its supporting bone. The overall quality and relative maturation of alveolar bone supporting a rat maxillary molar is shown by a microradiographic image of a histologic section (Figure 26-20, A). If the same section is viewed with fluorescent light, the pattern of osteogenic activity in the bone directly supporting the PDL is visible. Sharp labels mark lamellar bone, and diffuse labels indicate woven bone. Extensive turnover (remodeling) of the alveolar process is shown by uptake of internal labels (Figure 26-20, B). These data reveal that the entire alveolar process responds to tooth movement. Uncoupled anabolic and catabolic modeling occurs along bone surfaces that border the periosteum and PDL. Remodeling (coupled foci of bone resorption and formation) is the process of internal turnover and adaptation.
To a limited extent the temporal fossa can adapt to growth and functional loading, primarily in an anteroposterior direction, but the principal site of skeletal growth and adaptation is the mandibular condyle. In one study, multifluorochrome labeling and microradiography were used to compare bone in growing adolescent rabbits (Figure 26-21) with that in adult female rabbits who had completed growth (Figure 26-22). The adolescent primary spongiosa, the layer of endochondral bone immediately beneath the articular cartilage, is predominantly woven bone (marked by the diffuse labels in Figure 26-22). More inferiorly, the primary spongiosa is remodeled to secondary spongiosa (broad, distinct labels). Progressing deeper into the secondary spongiosa (trabecular bone), continuing remodeling of lamellar bone is shown by the sharp labels (see Figure 26-21). This progressive pattern of bone modeling and remodeling is characteristic of the skeletal mechanism of long bone growth.
In contrast, the nongrowing condyles of adult animals have a much thinner subchondral plate composed primarily of woven bone (see Figure 26-22). The supporting metaphysis is composed entirely of secondary spongiosa. Bone label uptake documents a high rate of remodeling of lamellar bone.
These data suggest that the mandibular condyle has a high rate of remodeling consistent with heavy functional loading. All things considered, the substantial histologic variance of functioning condyles in adolescent and adult animals indicates that the TMJ is highly adaptable. However, the presence of woven bone and diffuse labels in the thin subchondral plate of adults suggests that the mandibular condyle may be fragile and susceptible to degenerative changes if overloaded. Nevertheless the high rate of physiologic activity in the mandibular condyle of young and old animals may explain the remarkable ability of this joint to heal and even regenerate after injury (see Figure 26-14).
Microindentation, Backscatter Imaging, and Microcomputed Tomography
Huja et al.21 developed a microindentation method for determining the material properties of bone in a block specimen and demonstrated that the lamellar bone within 1 mm of the surface of an implant is more compliant than the supporting bone of the jaw. Polarized microscopy demonstrates the more irregular collagen pattern of the compliant lamellar bone near the interface (Figure 26-23). Backscatter emission imaging19 recently has been refined as a high-resolution method for assessing the bone mineral density and surface topography patterns of the osseous interface of dental implants (Figure 26-24). In another important technologic advancement, Yip et al.20 developed a special tuning sequence for the microcomputed tomography that allows three-dimensional detection of bone mineral density patterns to a resolution of 5 μm (Figure 26-25). Furthermore, this exciting new method can detect bone remodeling foci within intact specimens (Figure 26-26) and can differentiate between primary and secondary lamellar bone along the metallic surfaces of endosseous implants. Collectively, these new methods have been valuable for assessing the material properties and mineral density of bone integrating endosseous implants that are used for orthodontic and dentofacial orthopedic anchorage. However, these advanced technologies offer the promise of considerably exceeding the capability of previous histologic methods for defining the adaptive response of the oral and craniofacial structures to therapeutic loads.
Autoradiography
Radioactive precursors for structural and metabolic materials can be detected in tissue by coating histologic sections with a nuclear track emulsion. By localizing radioactive disintegrations, one can determine the location of the radioactive precursors (Figure 26-27). Specific radioactive labels for proteins, carbohydrates, and nucleic acids are injected at a known interval before tissue sampling is done. Qualitative and quantitative assessment of label uptake is a physiologic index of cell activity. The autoradiographic labeling procedures most often used in bone research are 3H-thymidine labeling of cells synthesizing DNA (S phase cells) and 3H-proline labeling of newly formed bone matrix. Bromodeoxyuridine immunocytochemistry, a nonradioactive method of labeling S phase cells in vivo (Figure 26-28), shows promise of becoming an important bone cell kinetic method of the future.14
Nuclear Volume Morphometry
Measuring the size of the nucleus is a cytomorphometric procedure for assessing the stage of differentiation of osteoblast precursor cells. This method has been particularly useful for assessing the mechanism of osteogenesis in orthodontically activated PDLs (Figure 26-29). Preosteoblasts (C and D cells) have significantly larger nuclei than committed osteoprogenitor (A′) cells or their less differentiated precursors (A cells). The B cell compartment is a group of fibroblast-like cells that appear to have little or no osteogenic potential.28 Careful cytomorphometric assessment of the size of the nucleus (Figure 26-30, A) has proved to be an effective means of determining the relative differentiation of PDL and other bone-lining cells.
Classification of Bone Tissue
Orthodontic tooth movement involves a cytokine-mediated bone adaptation response similar to wound healing; therefore, tooth movement is a good experimental model for understanding the types of bone formed during the postoperative bone modeling and long-term remodeling response to bone manipulative therapy. The first bone formed is relatively immature woven bone (Figure 26-31). Woven bone is compacted to form composite bone (primary ostons) and subsequently is remodeled to lamellar bone. To appreciate the biologic mechanism of bone healing and adaptation, the practitioner must have knowledge of bone types.
Woven Bone
Woven bone varies considerably in structure; it is realtively weak, disorganized, and poorly mineralized. However, it serves a crucial role in wound healing by (1) rapidly filling osseous defects; (2) providing initial continuity for fractures, osteotomy segments, and endosseous implants; and (3) strengthening a bone weakened by surgery or trauma. The first bone formed in response to wound healing is the woven type. Woven bone is not found in the adult skeleton under normal, steady-state conditions; rather, it is compacted to form composite bone, remodeled to lamellar bone, or rapidly resorbed if prematurely loaded.8,29 The functional limitations of woven bone are an important aspect of orthodontic retention (see Figure 26-16), as well as postoperative healing of implants and orthognathic surgery segments.30
Lamellar Bone
Lamellar bone, a strong, highly organized, well-mineralized tissue, makes up more than 99% of the adult human skeleton. When new lamellar bone is formed, a portion of the mineral component (hydroxylapatite) is deposited by osteoblasts during primary mineralization (see Figure 26-15, D). Secondary mineralization, which completes the mineral component, is a physical process (crystal growth) that requires many months. Within physiologic limits, the strength of bone is related directly to its mineral content.24,26 The relative strengths of different histologic types of osseous tissue are such that woven bone is weaker than new lamellar bone, which is weaker than mature lamellar bone.30 Adult human bone is almost entirely of the remodeled variety: secondary osteons and spongiosa.7,8,24 The full strength of lamellar bone that supports an endosseous implant is not achieved until about 1 year postoperatively. This is an important consideration in planning the functional loading of an implant-supported prosthesis.
Composite Bone
Composite bone is an osseous tissue formed by the deposition of lamellar bone within a woven bone lattice, a process called cancellous compaction.6,31 This process is a rapid means of producing relatively strong bone in a short period.26 Composite bone is an important intermediary type of bone in the physiologic response to functional loading (see Figure 26-31), and it usually is the predominant osseous tissue for stabilization during the early process of postoperative healing. When the bone is formed in the fine compaction configuration, the resulting composite of woven and lamellar bone forms structures known as primary osteons. Although composite bone may be high-quality, load-bearing osseous tissue, it is eventually remodeled into secondary osteons.7,30
Bundle Bone
Bundle bone is a functional adaptation of lamellar structure to allow attachment of tendons and ligaments. Perpendicular striations, called Sharpey’s fibers, are the major distinguishing characteristics of bundle bone. Distinct layers of bundle bone usually are seen adjacent to the PDL (see Figure 26-31) along physiologic bone-forming surfaces.32 Bundle bone is the mechanism of ligament and tendon attachment throughout the body. First-generation blade implants were thought to form a ligamentous attachement to bone, which was deemed a pseudoperiodontium. However, histologic studies could not demonstrate any bundle bone attaching fibrous conective tissue to bone at the interface. Because the fibrous tissue encapsulation had no physiologic role, it was actually scar tissue, which was equivalent to a nonunion in a failed facture repair.
SKELETAL ADAPTATION: MODELING AND REMODELING
Skeletal adaptation to the mechanical environment is achieved through changes in (1) bone mass, (2) geometric distribution, (3) matrix organization, and (4) collagen orientation of the lamellae. In addition to these adaptive mechanisms that influence bone formation, the mechanical properties of osseous structures change as a result of maturation, function, aging, and pathologic processes. A few physiologic and pathologic examples are (1) secondary mineralization, (2) mean bone age, (3) fatigue damage, and (4) loss of vitality (pathologic hypermineralization).33
Trabecular and cortical bone grow, adapt, and turn over by means of two fundamentally distinct mechanisms: modeling and remodeling. In bone modeling, independent sites of resorption and formation change the form (shape, size, or both) of a bone. In bone remodeling, a specific, coupled sequence of resorption and formation occurs to replace previously existing bone (Figure 26-32). The mechanism for internal remodeling (turnover) of dense compact bone involves axially oriented cutting and filling cones (Figure 26-33).6 From an orthodontic perspective the biomechanical response to tooth movement involves an integrated array of bone modeling and remodeling events (Figure 26-34, A). Bone modeling is the dominant process of facial growth and adaptation to applied loads such as headgear, rapid palatal expansion, and functional appliances. Modeling changes can be seen on cephalometric tracings (see Figure 26-34, B), but remodeling events, which usually occur at the same time, are apparent only at the microscopic level. True remodeling usually is not imaged on clinical radiographs,34 but can be detected with clinical scintillation scans. Constant remodeling (internal turnover) mobilizes and redeposits calcium by means of coupled resorption and formation: bone is resorbed and redeposited at the same site. Osteoblasts, osteoclasts, and possibly their precursors are thought to communicate by chemical messages known as coupling factors. Transforming growth factor β is thought to be a coupling factor.35
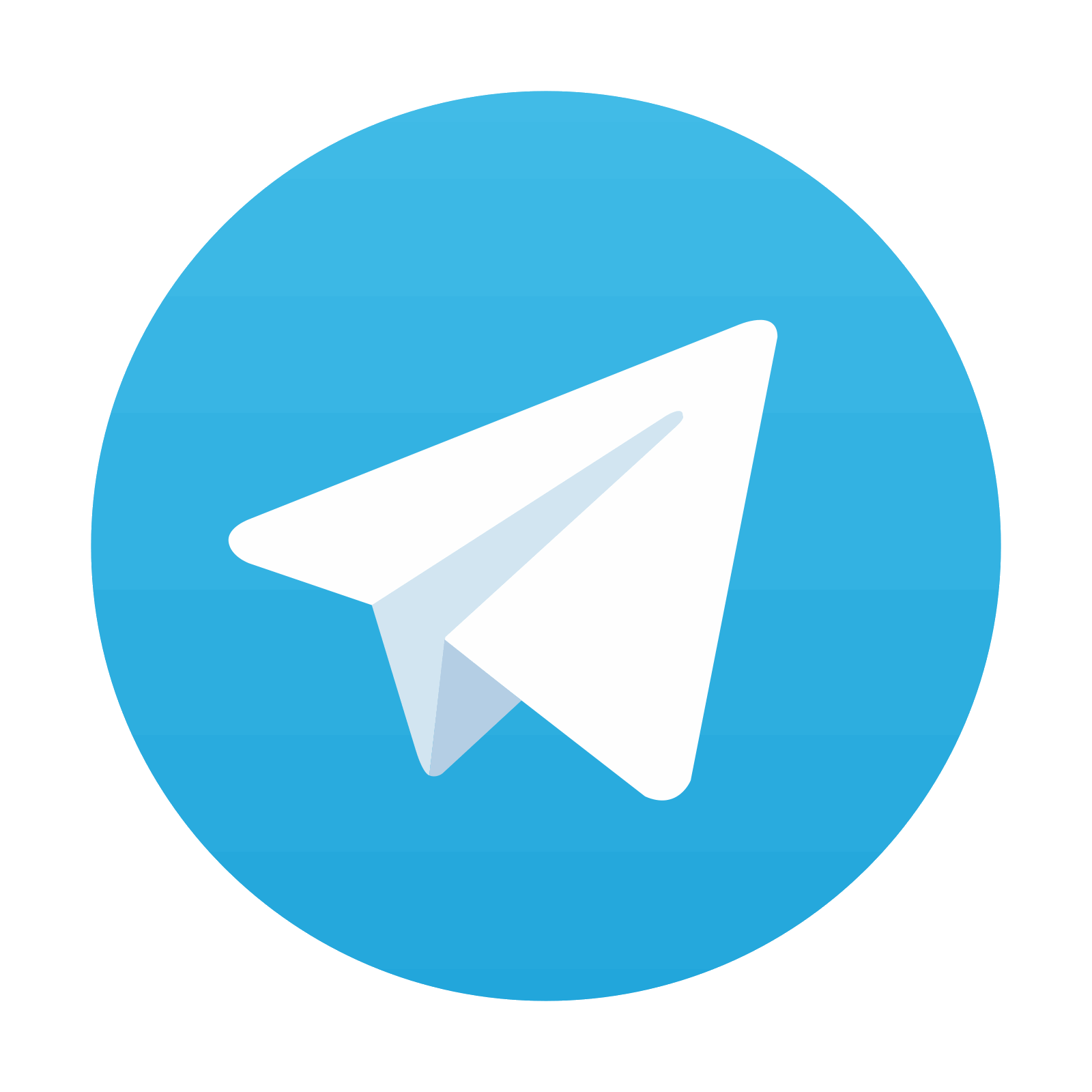
Stay updated, free dental videos. Join our Telegram channel

VIDEdental - Online dental courses
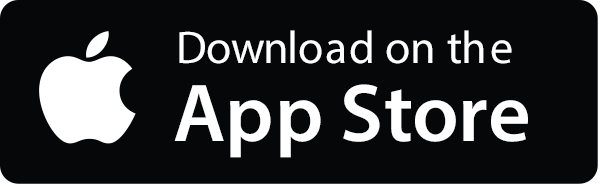
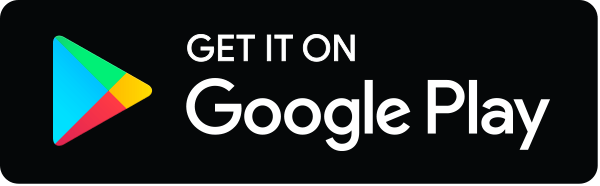